Fig. 15.1
A consistent relationship between increases in pulmonary artery pressures and cardiac output during exercise. Echocardiographic estimates of pulmonary artery pressures (PAP)—panels (a) and (b)—demonstrate a near-linear relationship between increases in PAP and cardiac output (CO). These findings have been validated in untrained and trained subjects using direct invasive pulmonary artery pressure measures (panels (a) & (c)—authors’ own unpublished data and panel (b) adapted with permission from Argiento et al. [18])
Moreover, the increase in PAP seems disproportionate to the more moderate increase in the systemic vascular pressure. We sought to assess this seemingly disproportionate ventricular load using a combination of magnetic resonance and echocardiographic imaging at rest and during exercise to quantify RV systolic wall stress, as compared with that of the LV [19]. Using the simple construct of the LaPlace relationship, we found that during exercise the increase in both pressure and volume was greater for the RV, while the increase in wall thickness was relatively less than that of the LV. As a result, the estimated RV wall stress estimates increased 125 % during exercise as compared with a modest 14 % increase in LV wall stress [19]. Thus, it appears that the stress, work, and metabolic demands placed on the RV during strenuous exercise are relatively greater than that of the LV. The hemodynamic stress on the RV can be visualized during exercise using novel techniques such as exercise cardiac magnetic resonance imaging (CMR). Video 15.1 provides a comparison between biventricular function in a nonathlete, an athlete, and a patient with pulmonary hypertension. The RV dilation and septal shift during exercise can be appreciated as can the similarities between the exercise hemodynamic stressors in the athlete and the patient with pulmonary hypertension.
RV Structural Changes
The athlete’s heart phenotype is usually described as a symmetrical increase in the dimensions of all four cardiac chambers. The nature and extent of cardiac remodeling is defined by a number of factors, some of which remain somewhat speculative (Fig. 15.2). It is likely that genetic and other factors modify remodeling but certainly the recurrent hemodynamic stressors imposed during regular training and competition are a major influence. As was first documented in the seminal work by Morganroth et al. [22] and later validated by others [23, 24], ventricular mass is increased after both strength and endurance exercise but endurance exercise training results in greater chamber dilation as a result of volume load. Thus, changes in ventricular structure reflect the hemodynamic load imposed and this would imply that disproportionate RV remodeling may occur as a result of the greater hemodynamic load posed on the RV.


Fig. 15.2
Training induced changes in cardiac morphology. Differences in exercise hemodynamic stressors determine cardiac morphology in endurance and strength trained athletes with important genetic, training-related and other modifying influences which remain imprecisely characterized
There are relatively few studies which have compared the extent to which exercise remodeling affects the RV relative to the LV, and the available evidence is conflicting. The structural effects of regular endurance training on the right heart are superficially similar to the effects on the left heart with myocardial hypertrophy resulting in increased right ventricular mass and increased right ventricular volume. In an older adult cohort, increased right ventricular volume and mass are associated in a fairly linear fashion with the amount of self-reported exercise performed [25], independent of other clinical variables and independent of LV mass and volumes. In endurance athletes, the degree of left and right ventricular dilation has been reported to be similar [26–29] reflecting the similar degrees of volume loading, although recent work suggests that the right ventricle may enlarge to a slightly greater extent [19]. As a result of the increased RV volume and a normal stroke volume, a reduced resting right ventricular ejection fraction is frequently observed in highly trained athletes.
One study in track athletes demonstrated that the right ventricular mass was increased when compared to nonathletes in both sprinters and marathon runners [30]. In the same cohort, in anaerobic power track athletes, more pronounced right ventricular dilation is seen on CMR scanning than in the marathon runners.
Furthermore, some insights may be gained from animal experiments based on an induced aortocaval fistula in pigs [31]. This intervention leads to a chronic cardiac high-output state with an increase in volume and pressure load somewhat akin to exercise. At 3 months after the surgery, there was a disproportionate increase in RV stroke work index relative to that of the LV (+216 % vs. +70 %), RV fibrosis, and the development of RV dysfunction. As compared with the more “physiological” LV hypertrophy characterized by myocyte length increase and increased local production of insulin-like growth factor, the RV showed more pronounced hypertrophy, increase in both myocyte length and diameter, and associated increased collagen deposition [31].
Thus, most forms of exercise result in increases in right ventricular mass and volume. The degree of increase appears to relate to the amount of exercise performed and has been observed both in cohorts who are already training and following training of sedentary individuals. The degree of right ventricular remodeling in endurance athletes appears to be slightly greater than that seen for the left ventricle.
RV Functional Changes
There are scant data to guide our understanding of RV functional changes as a result of athletic training. Furthermore, assessment of RV function is confounded by structural changes because the RV enlargement that constitutes part of the athlete’s heart phenotype also affects measures of RV function. In a larger heart, variables measuring motion tend to be larger [32] while variables measuring deformation and ejection fraction tend to be lower [33]. Available data need to be interpreted within this context. For example, D’Andrea et al. observed that tricuspid annular motion was greater in athletes than in nonathletes [34] while, in contrast, Teske et al. [35] and our own data [36] suggest that resting measured variables of strain and strain rate tend to be lower in endurance athletes relative to controls. However, during exercise these values tend to normalize suggesting that contractile reserve is normal in athletes as might be expected given the remarkable cardiac outputs generated [36]. In a relatively large magnetic resonance imaging study of over 300 subjects, RV ejection fraction was lower in endurance athletes when compared with nonathletes (50 vs. 52 % in men and 53 vs. 55 % in women) [37]. Thus, the interpretation of many of these studies is problematic with some authors concluding that RV function is supra-normal and others that RV function is impaired. However, the extent to which these functional measurements represent, true changes in myocardial contractility, is difficult to determine and, moreover, we would argue that it is most important to determine myocardial function during exercise when its ability to respond to increased demand can be appraised.
The combination of structural and functional changes in the endurance athlete’s heart can be profound and can lead to clinical difficulties in determining whether the extent of RV remodeling can be considered normal for an athlete or may be an indication of evolving pathology. Figure 15.3 and Video 15.2 provide examples of the extent of RV remodeling in four elite cyclists from a single professional team.


Fig. 15.3
Examples of cardiac morphology in four elite cyclists. Refer also to Video 15.1 to observe cardiac function in these athletes. The images exemplify the RV dilation, hypertrophy, and hypertrabeculation which can be commonly seen amongst high-level athletes
The Importance of the RV and Pulmonary Vasculature to Athletic Performance
Right ventricular function and the state of the pulmonary vascular bed appear to be important determinants of exercise capacity. While this has been long appreciated in disease states such as pulmonary arterial hypertension where right ventricular dysfunction has been shown to be a marker of both exercise capacity and poor prognosis [38–43], the same cannot necessarily be extrapolated to healthy athletes. Rather, it seems that increased RV dilation is associated with improved exercise performance in athletes.
It has been shown that RV volume is an important correlate of peak exercise oxygen consumption (VO2peak) [12]. However, given that changes in cardiac structure are relatively balanced between the RV and LV, it is difficult to determine whether changes in RV structure and function are any more important in predicting exercise capacity than measures of the LV or whole heart [44]. Interestingly, the two studies that have investigated the relationship between ventricular volumes and VO2peak have observed a slightly stronger correlation between RV than LV volumes. However, with such significant collinearity between ventricular measures, it is most reasonable to conclude that it is the global increase in cardiac size which best determines exercise capacity.
As has been discussed in the preceding sections, the RV and pulmonary circulation are placed under disproportionate stress during exercise which raises the intriguing hypothesis that the pre-systemic circulation may be an important determinant of exercise capacity. However, assessment of the pulmonary circulation is difficult using noninvasive measures and particularly so during exercise. A number of studies have identified a novel noninvasive surrogate which appears to describe pulmonary vascular physiology. Eldridge et al. [45] observed that agitated saline contrast filled the RV, but that the bubbles were too large to pass through the pulmonary circulation at rest. During exercise, however, the bubbles passed through the pulmonary circulation and could be visualized with echocardiography passing into the left-sided heart chambers (Fig. 15.4). The authors concluded that this represented passage through larger pulmonary vessels and hypothesized that this may be a physiologically advantageous trait enabling higher flows at lower vascular pressures. La Gerche et al. validated this theory by demonstrating that those subjects with greater amounts of contrast in the left heart during exercise had higher cardiac outputs, lower pulmonary artery pressures, and higher pulmonary vascular compliance than did those with few bubbles passing through the pulmonary circulation [20]. It was observed that the transit of bubbles through the pulmonary circulation occurred to a similar extent in athletes and nonathletes, implying that it is a non-trainable characteristic, and that it was associated with greater VO2peak and better exercise-induced augmentation of RV function.


Fig. 15.4
Transpulmonary passage of agitated contrast. Echocardiographic apical four chamber views at rest (left panel) and at exercise (middle and right panels) after the injection of agitated contrast, showing appearance in the left heart chambers after 4–5 beats at moderate exercise, and more so at intense exercise. The contrast can be seen entering the left atrium via the pulmonary veins (arrowheads) during exercise. This phenomenon has been associated with enhanced pulmonary vascular function and greater cardiac outputs during exercise
The exact mechanism underlying the transpulmonary passage of agitated contrast (PTAC) remains speculative. It has been suggested that it represents recruitable arteriovenous shunts [46] whereas we and others have argued that it is more likely a reflection of microvascular recruitment and distensibility. Using lung diffusing capacities of carbon monoxide and nitric oxide, the group of Lalande and Naeije demonstrated that the transpulmonary passage of agitated contrast was associated with greater pulmonary arteriolar distensibility and capillary recruitment [47]. Furthermore, they again found an association between transpulmonary contrast and VO2peak confirming that pulmonary vascular and RV function during exercise may be an important constraint during exercise and that agitated contrast may be a useful surrogate for identifying those with better pulmonary vascular reserve.
Beyond VO2peak, there are very few studies that have investigated a relationship between exercise performance and cardiac variables. Intriguingly, Bernheim et al. recently studied the relationship between cardiac morphology and the time taken to complete an ultra-endurance triathlon and found a relatively strong inverse relationship between time and RV volumes; that is, the larger the RV the faster the athlete [48]. Further confirmation is required for each of these lines of inquiry, but current evidence suggests that there may indeed be a relationship between exercise performance and cardiovascular function and that variables pertaining to the RV and pulmonary circulation may be most instructive.
Evidence of Adverse Effects of Endurance on Cardiac Structure and Function
As has been detailed, exercise training is associated with changes in cardiac structure and function which tend to favor the RV and which are associated with improved exercise performance. However, in contrast to these seemingly beneficial physiological adaptations, there is evidence to suggest that some of the exercise-induced changes may be associated with acute and chronic cardiac damage and that in a small number of athletes this may predispose individuals to atrial and ventricular arrhythmias. Concern regarding the potential for proarrhythmic remodeling in athletes stems from the observations of cardiac damage following endurance sporting events, an increased prevalence of some arrhythmias amongst endurance athletes and evidence of chronic structural changes or ‘scar’ within the myocardium of some veteran endurance athletes. Each of these factors will be discussed in detail.
Biomarkers in Endurance Exercise
Studies of endurance athletes following competitive events in different disciplines and of different durations have shown evidence of acute myocardial injury based on the transient elevation of biomarkers following these events. Events have included ultra-endurance triathlon, ultra-endurance cycling events, and marathon running. Biomarkers studied have included troponin T, troponin I and B-type natriuretic peptide. The first study examining this issue in marathon runners was published in 1981 and based on monitoring of the CK-MB isoenzyme [49]. Elevated CK-MB levels were seen following marathon running, but this work was confounded by the fact that CK-MB may be elevated by skeletal muscle injury, an almost universal outcome following a marathon.
The first evidence using more specific biomarkers appeared in 1999, when Rifai and co-workers studied 23 participants competing in an ultra-endurance triathlon and found elevated levels of cardiac troponin T and CK-MB in the athletes at the conclusion of the event [50]. They also observed changes in left ventricular function and concluded that ultra-endurance exercise may cause myocardial damage, although it was unclear from this study, which did not follow up on the participants, whether the damage was transient or permanent. Other studies also showed a similar increase in cardiac troponin levels in cycling [51, 52], triathlon [53, 54], and marathon running [55], all events with an average duration of 4 h. A meta-analysis suggested that elevated cardiac troponin levels occur in approximately 50 % of endurance exercise event participants [56]. In all studies, cardiac enzyme levels returned to the normal range within a few days of the exercise.
The consistent finding of elevated markers of cardiac injury, but rapid normalization prompted a debate regarding the origin, mechanisms, and significance of these biomarkers. Neilan observed differences in the frequency of biomarker elevations in marathon runners, with less trained individuals being more likely to have high troponin T levels [57]. In the meta-analysis of Shave et al., elevated levels were more common after shorter events, thought to be due to the higher intensity at which the events are performed [56]. In those competing in longer events, there does not appear to be a clear relationship with training levels [58]. Some authors have argued that the elevation of cardiac troponin levels, generally considered to be evidence of cardiomyocyte necrosis, was caused by alternative mechanisms. Some have argued that increased cardiomyocyte membrane permeability due to myocardial stress results in diffusion of cytosolic troponin into the extracellular space [56]. Others have argued that the kinetics of troponin release with rapid normalization is suggestive of altered myocyte metabolism rather than necrosis [59].
It appears that both exercise duration and intensity are important determinants of the occurrence and magnitude of troponin release during exercise.
Acute Changes in RV Function with Exercise
Acute changes in cardiac function have been repeatedly demonstrated following endurance sporting events. Evidence regarding LV function following endurance exercise is substantive but frequently conflicting [60]. A meta-analysis of 23 studies concluded that intense endurance exercise was associated with a modest decrease in LV ejection fraction (–2 %) which was at least partially attributable to changes in cardiac loading in the post-race setting. In contrast, a number of recent studies have assessed RV function post-endurance exercise and have consistently reported decrements in RV function which are far more substantive than those observed for the LV. Relative to baseline measures, studies have reported reductions in simple geometric measures of RV function such as fractional area change and tricuspid annular displacement [57, 58, 61, 62]. Using M-mode studies two decades ago, Douglas et al. demonstrated that the RV dilated following an ultra-endurance triathlon whereas the LV dimensions were unchanged [63] and this observation has recently been replicated using 2D and 3D echocardiography[58, 62]. The result of the RV dilation combined with pericardial constraint is that the interventricular septum is pushed toward the LV resulting in an increase in the LV eccentricity index [58, 62]. It has been hypothesized that this “squashing” of the LV during early diastole may explain some of the observed changes in LV diastolic variables.
It may be argued that echocardiographic assessment of RV function has significant limitations. However, studies using cardiac magnetic resonance imaging (CMR) post-IEE have confirmed the same differential effects with no change in LV function and a considerable reduction in RVEF [64, 65]. Finally, while multiple studies have documented that there is no relationship between biomarkers of cardiac injury and changes in LV function [66], two recent studies have documented moderately strong inverse correlations between the decrease in measures of RV function and the increase in release of troponin and B-type natriuretic peptide [57, 58]. Thus, it would seem that the RV is potentially the “Achilles’ heel” of the endurance athlete which is disproportionately fatigued or injured following endurance exercise.
Arrhythmias in Athletes
While athletes are not immune to the disturbances of cardiac rhythm seen by nonathletes, palpitations and arrhythmias are a common problem observed in sports cardiology practice. There is now reasonably compelling evidence that some cardiac arrhythmias are associated with long-standing endurance training. Almost every study conducted in endurance athletes of middle age or older athletes has observed an increased prevalence of atrial fibrillation (AF) as compared with nonathletic referents [67–74]. In a meta-analysis, Abdulla et al. determined a 5.3-fold relative risk for the development of AF amongst studies matching athletes (almost exclusively male) to nonathletic controls [6].
Evidence for an excess of other cardiac arrhythmias is less well established. Biffi et al. reported that ventricular ectopic beats were common amongst athletes but this was a benign and potentially reversible phenomenon as long as underlying cardiac disease was excluded [75, 76]. In contrast, Heidbuchel et al. observed a high incidence of major arrhythmic events (39 %) including sudden cardiac death (20 %) amongst 46 athletes, followed for 5 years, who presented with frequent ventricular ectopy or non/sustained ventricular tachycardia [77]. Somewhat surprisingly, in 89 % of cases the arrhythmias arose from the RV and were frequently associated with functional and/or structural changes of the RV. In a subsequent study by the same group, the presence of right ventricular arrhythmias was associated with increased right ventricular size and decreased right ventricular systolic function when compared to athletes without ventricular arrhythmias and nonathletes [78]. In these studies there was only one athlete with a family history suspicious for an inherited disease process suggesting that the RV remodeling was unlikely to be explained by arrhythmogenic right ventricular cardiomyopathy (ARVC) (see Chap. 16). Thus this group of endurance athletes appeared to have an unexpectedly high rate of arrhythmias arising from the right ventricle and unexpected right ventricular dysfunction.
Evidence of Chronic Myocardial Changes Affecting the RV
An accumulating body of circumstantial evidence suggests that there may be some overlap in the spectrum from physiological to pathological hypertrophy such that a small amount of fibrosis may accompany more profound cardiac remodeling associated with lifelong endurance training. The concept of purely physiological remodeling in response to exercise training implies that myocyte hypertrophy and hyperplasia is stimulated in response to a hemodynamic load and is downregulated once that stimulus is removed [79]. Thus we would expect that cardiac size would return to normal in athletes who de-train. However, Pelliccia et al. prospectively followed 40 elite male athletes and found that while cardiac dimensions did decrease with detraining, substantial cavity dilation persisted in nine athletes (22 %) [80] and a number of other studies have reported enlarged cardiac dimensions amongst retired endurance athletes and related the extent of these changes to the development of arrhythmias [68, 72, 81, 82]. Thus, speculation has arisen that this persistent cardiac enlargement may reflect a degree of interstitial fibrosis and this hypothesis has recently been evaluated using CMR combined with gadolinium contrast. Cell necrosis and fibrosis lead to leaking of gadolinium into the extracellular space and this can then be identified using gradient-echo inversion recovery imaging as a bright signal contrasting with the normal myocardium which appears black (Fig. 15.5). This delayed gadolinium enhancement (DGE) technique has been investigated in small cohorts of athletes and while it appears that DGE tends to be absent in athletes with modest training histories [64, 65, 83], four recent studies have each reported DGE in 12–50 % of extensively trained veteran athletes [58, 84–86]. La Gerche et al. identified DGE in 5 of 39 well-trained endurance athletes, and found that those with DGE had a more extensive history of training and had greater cardiac dimensions, particularly of the RV [58]. The patches of DGE have tended to be very small and clustered around the septum and RV insertion points, a region which may be subjected to local mechanical stress due to RV pressure afterload, in a manner similar to pulmonary hypertension [87, 88].


Fig. 15.5
Delayed gadolinium enhancement in highly trained athletes; evidence of exercise-induced myocardial scar? Images of five athletes in whom focal delayed gadolinium enhancement (DGE) were identified in the interventricular septum (indicated with arrows) as compared with an athlete with a normal study (top left). Reproduced with permission from La Gerche et al. [58]
Thus, it may be concluded that the majority of evidence that raises concern of cardiac damage secondary to extreme exercise tends to point toward the RV as the site of greatest injury. Acute post-race changes, chronic structural changes and proarrhythmic remodeling all tend to favor the RV more than the LV. Adding support to this concept from the animal domain, Benito et al. instituted a strenuous 18-week treadmill running regime in young rats estimating that this was the equivalent of 10 years of endurance exercise training in humans [89]. As compared with the sedentary control rats, there was an increase in atrial and right ventricular inflammation/ fibrosis in the “marathon rats” and, perhaps most importantly, this was associated with a greater potential for inducible ventricular arrhythmias (42 % vs. 6 %, p = 0.05). Once again, it is notable that just as in humans, there is a striking predilection for effects on the RV, as opposed to the LV.
Possible Mechanisms of RV injury
The available evidence supports the concept that endurance exercise may cause acute injury to the right ventricle and in some cases there may be more permanent changes in right ventricular structure and function. The mechanism of exercise-induced right ventricular dysfunction is not completely understood. A number of candidate mechanisms have been proposed and investigated to varying extents. A prime candidate is the hemodynamic stress under which the right heart is placed during activity. There is evidence that the increase in hemodynamic stress on the right ventricle during exercise is significantly greater when compared to the stress to which the left ventricle is subjected. Other possibilities which have been proposed include myocardial ischemia, possibly mediated through the effects of vasoconstrictor cytokines or elevated right ventricular end-diastolic pressures, the effects of differing training regimens or possibly through genetic susceptibility factors.
Hemodynamic Stress
As discussed in detail earlier in this chapter, evidence suggests that RV pressures, wall stress, and work all increase disproportionately to that of the LV during intense exercise. This is likely to manifest as greater RV injury regardless of the specific factors which cause exercise-induced myocardial injury. There are multiple candidate mechanisms including substrate deficiency, ischemia, β-adrenoreceptor desensitization, accumulation of metabolic toxins and oxidative stress. All of these may be expected to affect the RV given the greater hemodynamic stress on the pre-systemic circulation.
Training Regimen
It is likely that exercise intensity, exercise duration, and the degree of training are all important in the genesis of exercise-induced right ventricular injury. Some evidence supporting this premise comes from the studies discussed earlier which have documented changes in myocardial structure amongst highly trained veteran athletes but not amongst younger or less-trained athletes. We have also previously promoted the hypothesis of “over-training” of the heart (Fig. 15.6). Like over-use injuries such as tennis elbow or Achilles’ tendinopathy, intense training and lack of adequate recovery increase the risk of injury. In the heart this may manifest as small patches of fibrosis and an increased risk of arrhythmias.


Fig. 15.6
Healthy training vs. over-training of the heart. Healthy training with balanced exercise and recovery results in physiological remodeling in which enhanced cardiac structure and function enable greater cardiac performance during exercise. On the other hand, we propose that excessive exercise (training which is too intense and/or recovery that is too short) may cause cardiac injury and proarrhythmic remodeling which predominantly affects the right ventricle. Reproduced with permission from Heidbuchel et al. [102]
Myocardial Ischemia
Whereas ischemic injury at a microscopic and microcirculatory level cannot be excluded as a cause of exercise-induced right ventricular injury, in most published series of athletes with elevated cardiac biomarkers following exercise, acutely abnormal cardiac function following exercise, chronic right ventricular abnormalities or right ventricular arrhythmias, the presence of epicardial coronary artery disease has not been felt to be an important factor. The normal changes of acute myocardial infarction have not been present on ECG following endurance events, functional abnormalities have not been seen in a coronary artery distribution and typical changes of myocardial infarction have not been seen on CMR [90]. It is possible that variations of other factors such as myocardial capillary density may predispose some athletes to ischemia as a result of intense exercise. Capillary density has been shown to be an important determinant of myocardial ischemic damage in non-ST elevation myocardial infarction [91], possibly by increasing the distance between metabolizing myocytes and the capillaries supplying oxygen. Similar variability in right ventricular capillary density may play a role in exercise-induced right ventricular dysfunction, but has not been systematically studied.
Genetic Predisposition
One possible influence which needs consideration in the genesis of exercise-induced right ventricular dysfunction is genetic susceptibility. The phenotype in this condition shares similarities with arrhythmogenic right ventricular cardiomyopathy (ARVC—see Chap. 16), a genetically mediated cardiomyopathy associated with right ventricular enlargement, fibrosis, and susceptibility to ventricular arrhythmias, which may be lethal in some cases. ARVC is recognized as an important cause of sudden cardiac death during exercise, particularly in parts of Italy [92] and is actively screened for in young athletes in that country. A number of mutations in desmosomal components have been identified as causes of familial ARVC, although the five most common mutations seen in desmoplakin, desmoglein, plakophilin, plakoglobin, and desmocollin account for only 40 % of cases [93].
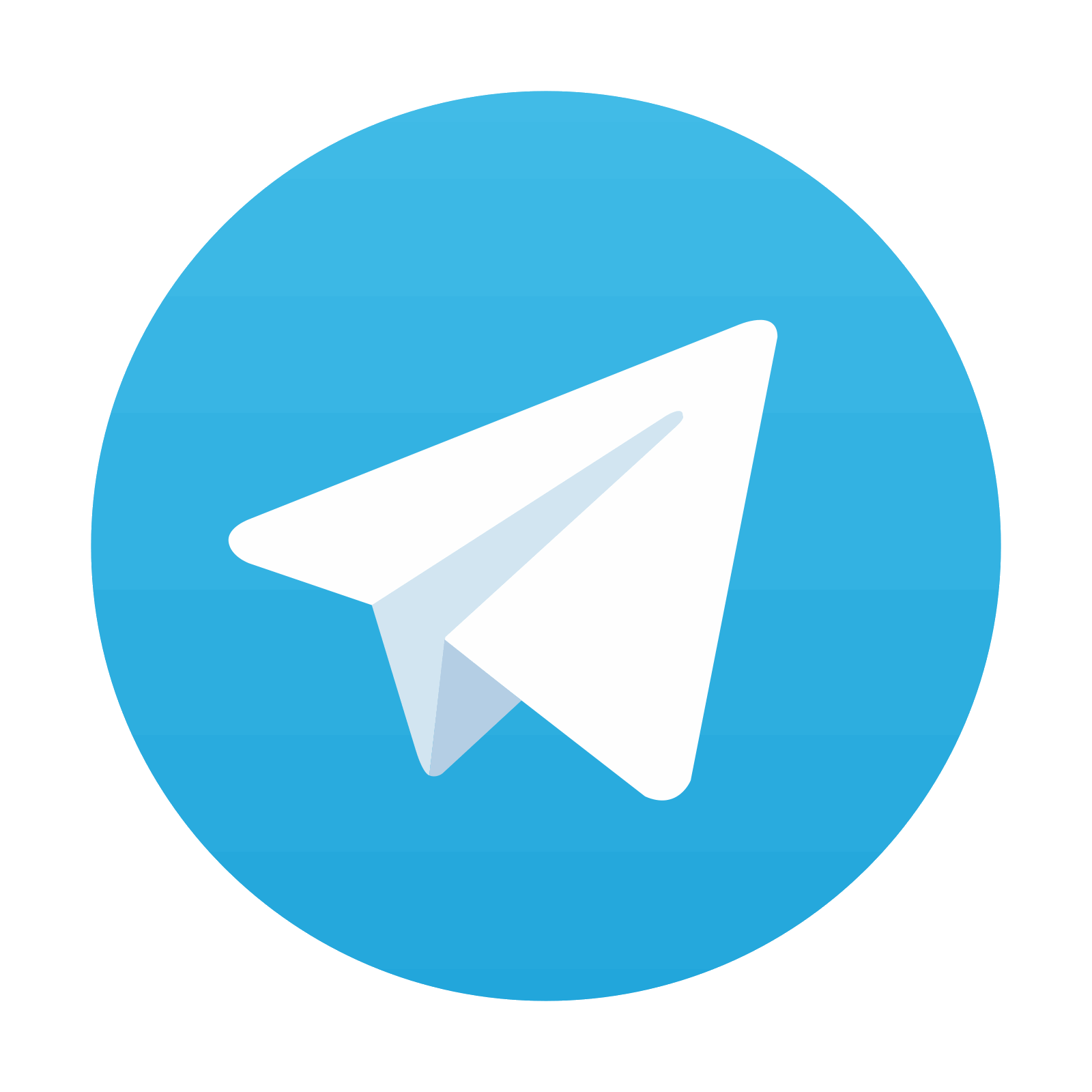
Stay updated, free articles. Join our Telegram channel
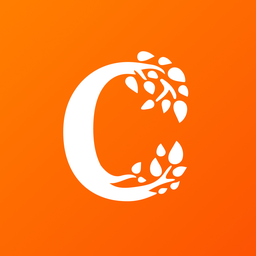
Full access? Get Clinical Tree
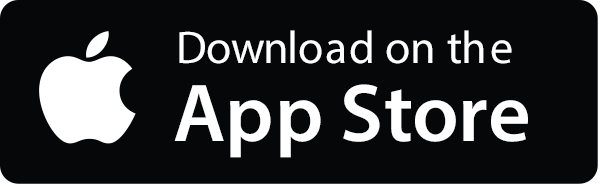
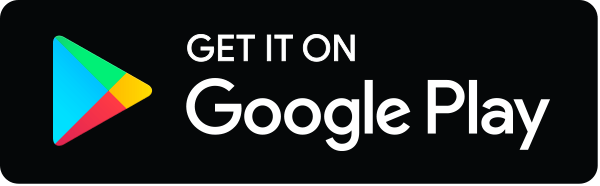