Fig. 6.1
Anatomy of the human heart showing the main structures. Blue regions indicate the right side of the heart, which pumps venous blood. Red regions indicate the left side of the heart, which pumps arterial blood. White arrows show the direction of blood flow
Each heartbeat is therefore reliant on a sequence of events that include mechanical contraction, as well as the opening and closing of the valves in the correct order. The outcome is an increase of pressure in the aorta, which acts to produce flow of blood around the systemic circulation. Figure 6.2 illustrates typical pressures and volume in the left side of the human heart during each beat. Atrial systole (contraction) produces a rise in LA pressure, filling the LV through the open mitral valve. The onset of ventricular systole results in pressure rise within the LV. Once LV pressure exceeds LA pressure, the mitral valve shuts, and when LV pressure exceeds pressure in the aorta the aortic valve opens. Blood is then ejected through the aortic valve, producing a pressure rise in the aorta. When LV pressure falls below aortic pressure the aortic valve closes, and when LV pressure falls below LA pressure the mitral valve opens.


Fig. 6.2
Cardiac cycle, showing changes in pressure and volume in different parts of the heart during two heart beats
6.1.2 Cardiac Cells and Tissue
The myocardium is a composite material, composed primarily of myocytes, fibroblasts and the extracellular matrix. Myocytes generate mechanical tension when stimulated electrically. Fibroblasts are connective tissue cells that act to regulate the extracellular matrix that supports the structures of the heart including the valves.
Cardiac myocytes are rod-shaped cells, 50–150 μm in length and 10–20 μm in diameter. When stimulated electrically, a myocyte generates tension in the direction of its long axis, and individual myocytes are connected end to end into fibres (Fig. 6.3a). The interface between adjacent cells has a characteristic stepped appearance and is called the intercalated disc. The intercalated discs contain gap junctions, which provide an electrical connection from one cell to its neighbour. Cardiac myocytes may have more than one nucleus, and may also branch, so that an individual cell may have more than two neighbours.


Fig. 6.3
Structure of cardiac tissue and myocytes. a Arrangement of cardiac myocytes and connections between them. b Internal structure of myocyte. c Diagram showing the contractile apparatus within a myofibril
In the ventricles, there is evidence that these fibres are also arranged into sheets, and this orthotropic structure contributes to the passive and active mechanical properties of the tissue (Nielsen et al. 1991). Figure 6.3a illustrates the typical arrangement of myocytes in tissue.
Myocytes are also composed of striated myofibrils (Fig. 6.3b). Each myofibril consists of chains of sarcomeres, each about 2 μm long, which are terminated at each end by a Z-disc. These Z discs provide an anchor for the thin filaments, which engage with thick filaments to generate tension (Fig. 6.3c). The mechanism of tension generation is described in more detail in Sect. 6.3.
A valve plane separates the electrically excitable atria and ventricles. It is composed of connective tissue and provides a mechanical anchor for the valves. The connective tissue is electrically inexcitable, and is penetrated only by the atrioventricular node (see below).
6.1.3 Myocardial Perfusion and Metabolism
The heart itself requires a supply of oxygenated blood in order for its metabolic needs to be met, and has its own system of arteries and veins. A branching network of coronary arteries is perfused from left and right branches, which connect to the aorta very close to the aortic valve. The main branches of the coronary arteries remain on the epicardial surface, and smaller vessels penetrate the myocardium. If a coronary artery develops a significant stenosis, then the region of myocardium perfused by that artery may become ischaemic. Ischaemia describes the changes in cell metabolism resulting from a reduction or interruption of the supply of oxygenated blood. These changes include altered electrical excitability, and reduced contractility. Prolonged or severe ischaemia resulting from complete blockage of a coronary artery will result in a myocardial infarction. Unless blood flow is restored quickly, the ischaemic region of myocardium will undergo irreversible cell death. Ultimately the region will become scar tissue, with impaired mechanical function.
Venous blood collects in the coronary sinus, which is located around the posterior of the heart, close to the valve plane. The coronary sinus drains into the right atrium.
6.2 Electrical Excitation
6.2.1 The Cardiac Action Potential
The mechanical contraction of cardiac cells and tissue is both initiated and synchronised by electrical excitation that originates in the sinus node, which acts as a natural pacemaker. The sequence of electrical excitation and recovery in a single cell is the cardiac action potential.
Cardiac myocytes are electrically excitable cells with a bilipid membrane that encloses the cell contents and acts as a barrier to the movement of ions. Embedded within the membrane are ion channels, pumps and exchangers, which have a permeability or conductance that depends on the potential difference across the membrane. An example of an ion channel embedded in the cell membrane is shown in Fig. 6.4. These ion channels, pumps and exchangers act to regulate the intracellular concentration of Na+, Ca2+ and K+.


Fig. 6.4
Cartoon of myocyte cell membrane, showing lipid biplayer, with embedded ion channel (elongated ellipsoids) admitting a single ion
At rest, the concentrations of Na+ and Ca2+ outside the cell exceed the concentration inside the cell, and the concentration of K+ inside the cell exceeds the concentration outside the cell (Table 6.1). At rest, the cell membrane is permeable to K+. A single K+ ion is therefore exposed to a concentration gradient, and so K+ ions tend to diffuse out of the cell. The effect of this diffusion is to establish a gradient in electrical potential because the K+ ions that diffuse out of the cell carry an excess positive charge. At equilibrium, the tendency to diffuse out of the cell is balanced by the opposing effect of potential difference. The corresponding voltage across the cell membrane at equilibrium is given by the Nernst equation
![$$ E = \frac{\text{RT}}{zF} { \log }_{e} \left( {\frac{{[{\text{K}}^{ + } ]_{o} }}{{[{\text{K}}^{ + } ]_{i} }}} \right), $$](https://i0.wp.com/thoracickey.com/wp-content/uploads/2017/11/A320302_1_En_6_Chapter_Equa.gif?w=960)
![$$ E = \frac{\text{RT}}{zF} { \log }_{e} \left( {\frac{{[{\text{K}}^{ + } ]_{o} }}{{[{\text{K}}^{ + } ]_{i} }}} \right), $$](https://i0.wp.com/thoracickey.com/wp-content/uploads/2017/11/A320302_1_En_6_Chapter_Equa.gif?w=960)
Table 6.1
Typical equilibrium concentrations of cations involved in the cardiac action potential
Ionic species | Intracellular concentration (mM) | Extracellular concentration (mM) | Nernst potential (mV) |
---|---|---|---|
Na+ | 10 | 140 | +70 |
Ca2+ | 0.0001 | 1.2 | +124 |
K+ | 140 | 5 | −90 |
where R is the gas constant (8.316 J K−1 mol−1), T is absolute temperature (body temperature is 310 K), z the valency of K+ ions (1), F the Faraday constant (charge carried by one mole of K+ ions, 96,484 C mol−1), [K+] concentration of K+ outside (o) and inside (i) the cell. For normal concentrations of K+, the Nernst potential is −90 mV, with the inside of the cell negatively charged relative to the outside. This is close to the resting potential of cardiac myocytes, which are polarised to a voltage typically between −80 and −90 mV. The K+ Nernst potential is not exactly equal to the resting potential because the membrane retains a very small permeability to Na+ ions, which acts to slightly reduce the resting potential.
When the potential difference across the cell membrane is perturbed so that it decreases below a threshold of around −65 mV (for example by an action potential in a neighbouring cell), voltage-gated ion channels open to admit Na+. There is a large gradient not only in Na+ concentration but also in electrical potential, which results in an influx of Na+ into the cell and a rapid depolarisation of the membrane potential towards the Nernst potential for Na+ ions, which is around +70 mV.
Almost immediately, the Na+ ion channels inactivate, shutting off further influx of Na+ ions. However, the change in membrane potential has two further consequences. First, the change opens voltage-gated Ca2+ ion channels, which admit Ca2+ into the cell. Second, the change also opens voltage-gated K+ ion channels, which results in an outward flow of K+ ions. Initially the influx of Ca2+ tends to balance the outflow of K+, but then the Ca2+ ion channels begin to close and the outflow of K+ dominates, leading to repolarisation of the cell to the resting potential. Computational and mathematical models have played an important role alongside experimental work in the discovery of these detailed physiological mechanisms, and this story is recounted by Noble and Rudy (2001).
The action potential is an all or nothing response, once the cell is depolarised to its threshold it will respond with a complete action potential. Once a cell begins an action potential, it will not respond to a further stimulus until is has repolarised. The cell is said to be refractory, and the interval between depolarisation and the time at which the cell can produce another action potential is called the refractory period. Typically, the refractory period is around the same as the action potential duration. Figure 6.5 shows time series generated from a computer model of the human atrial action potential, and includes both the action potential and the main currents that flow throughout the different phases.


Fig. 6.5
Cardiac action potential, with principal inward (Na+ and Ca2+), and outward (K+) currents shown
In cardiac tissue, cells are electrically connected by gap junctions, which enable the action potential to be passed from one cell to its neighbours. Since gap junctions are part of the intercalated discs, the action potential propagates faster along the long axis of cells than across the short axis.
6.2.2 Activation Sequence for Normal Beats
A normal heartbeat begins with the spontaneous depolarisation of pacemaking cells in the sinus node, located in the right atrium. In these specialised cells, there a small inward current gradually brings the membrane potential to threshold during the resting phase. The result is a series of spontaneous beats, and the interval between these beats is modulated by neural activity.
The normal beat initiated in the sinus node propagates through the left and right atrium, and into a further specialised region of tissue called the atrioventricular node. In the normal heart, the atrioventricular node provides the only pathway for an action potential to propagate through the fibrous tissue that separates the atria and ventricles, and acts as a mechanical support for the valves.
The atrioventricular node is linked to Purkinje fibres, which conduct the action potential very rapidly, and terminate throughout the ventricular tissue. The rapid propagation of the action potential through the Purkinje system ensures synchronised contraction of the ventricular chambers.
This sequence of electrical activation is shown in Fig. 6.6, which includes a representation of the action potential shape in each region.


Fig. 6.6
Cardiac action potentials in different regions of the heart
6.2.3 Origin of the Electrocardiogram
The sequence of electrical activation and recovery that acts to initiate and synchronies the mechanical activity of the heart can be observed on the torso surface as the electrocardiogram (ECG). The local electrical potential produced by action potentials in the tissue act to generate current flow within the torso, which behaves as a volume conductor. In turn, this current flow produces a potential on the body surface, and the time course of the potential reflects the sequence of activation and recovery in different parts of the heart.
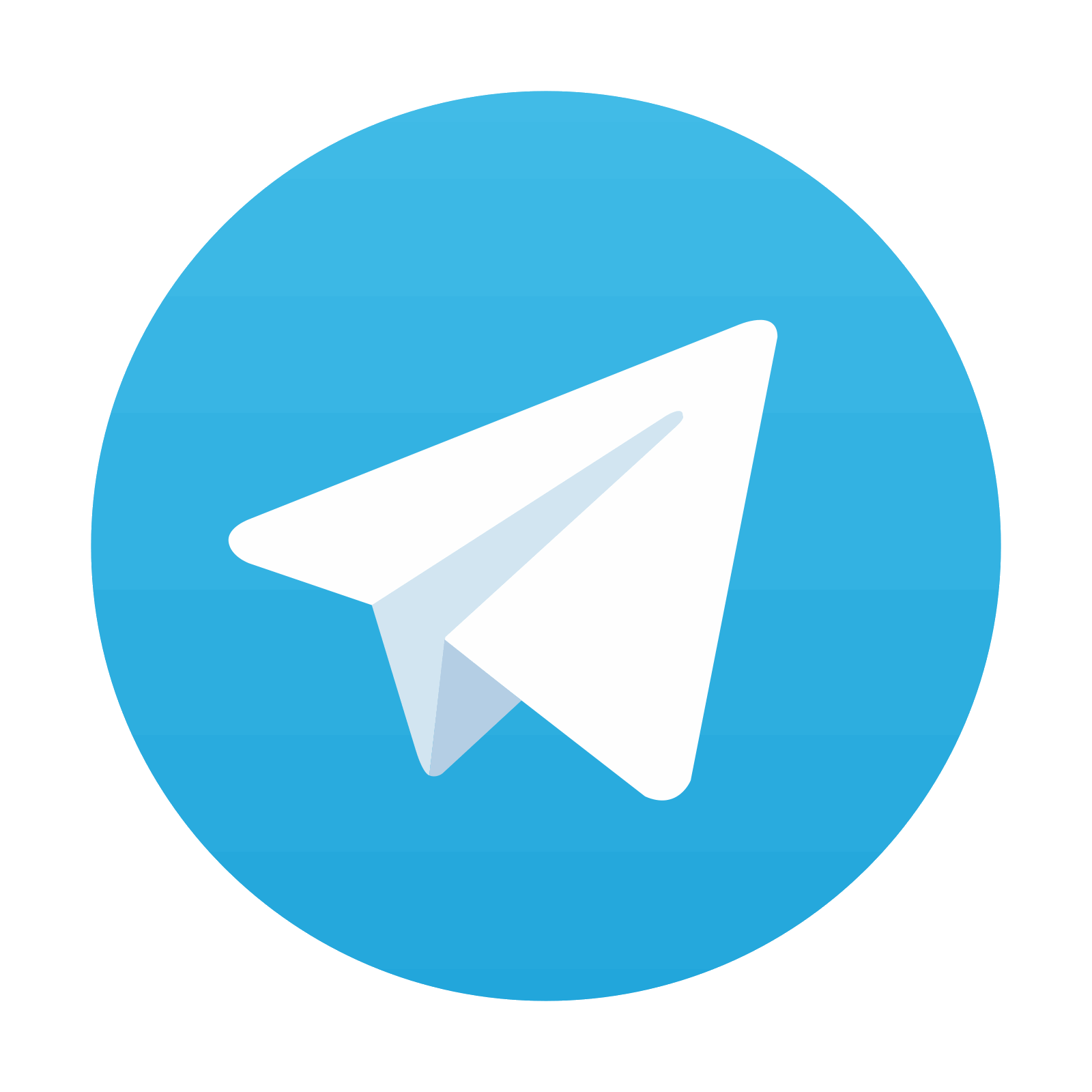
Stay updated, free articles. Join our Telegram channel
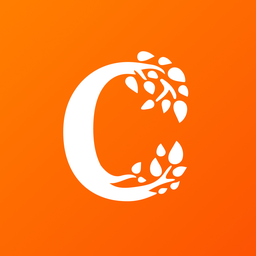
Full access? Get Clinical Tree
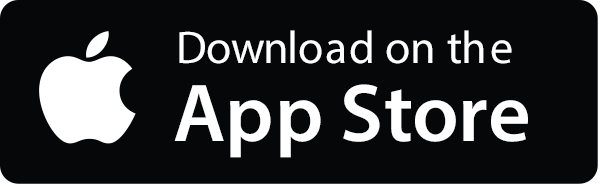
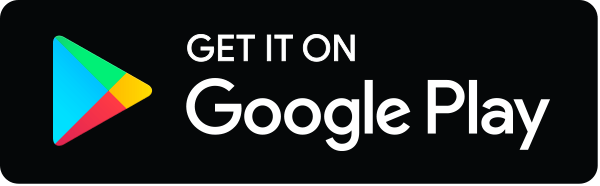