Fig. 8.1
Schematic of ex vivo organ engineering. Autologous cells are obtained by a biopsy from the eventual transplant recipient and expanded in ex vivo culture. A scaffold, either synthetic or an acellular lung, is manufactured and repopulated ex vivo by the usage of a bioreactor to create a functional tissue suitable for re-implantation
Synthetic scaffolds are one potential option and a number of different materials and manufacturing technologies have been evaluated for lung (Mondrinos et al. 2006, 2007; Lin et al. 2006; Andrade et al. 2007; Cortiella et al. 2006; Ingenito et al. 2010; Miller et al. 2010; Nichols and Cortiella 2008; Roomans 2010; Tsunooka et al. 2011). The porous structure of lung, like many other tissues, presents a unique manufacturing engineering challenge. Both additive (i.e., processes which generate final products layer-by-layer or unit-by-unit manufacturing) and subtractive processes (i.e., traditional manufacturing processes whereby material is removed to form the final product) are current areas of active research (Melchels et al. 2012). Additive processes, such as rapid prototyping three dimensional (3D) bioprinting techniques (Murphy and Atala 2014) are traditionally viewed as more advantageous for generating scaffolds with interconnected pores; however, subtractive processes such as porogen-forming techniques and sphere-templating have produced promising initial results (Bryant et al. 2007; Ling et al. 2014). Alternatively, hydrogels and electrospun scaffolds have also been proposed as potential scaffolds (Dunphy et al. 2014; Fischer et al. 2011). However, despite a variety of available state-of-the-art 3D printing and other technologies, these are so far unable to recapitulate the complex 3-dimensional architecture of the lung. Further, successful transplantation strategies and clinical use of synthetic lung scaffolds remain unknown.
An exciting new and active area of research involves the use of acellular lung scaffolds derived from cadaveric or failed transplant lungs. Acellular tissues are generated by removing cells from native organs while preserving the 3-dimensional macroarchitecture of the innate extracellular matrix (ECM) proteins (Badylak et al. 2012; Baiguera et al. 2012; Fishman et al. 2011; Haag et al. 2012; Haykal et al. 2012; Hinderer et al. 2012; Jungebluth et al. 2012a, b; Krawiec and Vorp 2012; Orlando et al. 2011; Ott et al. 2008; Totonelli et al. 2012; Wertheim et al. 2012). In the instance of lung, vasculature and airspaces are retained. This technique was originally described for lung tissue by Lwebuga-Mukasa et al. in 1986 where they utilized a decellularized rat lung to study type II alveolar epithelial (ATII) cell behavior on a native basement membrane (Lwebuga-Mukasa et al. 1986). Whole organ decellularization as a platform for organ regeneration was first described in heart in 2008 (Ott et al. 2008), and beginning in 2010, several groups described similar techniques in lung (Ott et al. 2010; Petersen et al. 2010; Price et al. 2010; Cortiella et al. 2010; Daly et al. 2012a; Wallis et al. 2012). The use of acellular lungs has since expanded beyond their usage in regenerative medicine and has become an incredibly powerful in vitro tool for studying cell–ECM interactions in a more in vivo-like culture system (Sokocevic et al. 2013; Wagner et al. 2014a, b; Booth et al. 2012; Zhou et al. 2014; Parker et al. 2014).
In this chapter, we will address some of the critical factors involved in the theoretical and practical considerations for use of decellularized whole lungs (alternatively referred to as acellular) for ex vivo lung regeneration. These include decellularization and recellularization procedures as well as the challenges which remain in creating a translationally feasible strategy for the clinic (Fig. 8.1).
8.2 Decellularization
8.2.1 Methods of Decellularization
Acellular biologic scaffolds have been created from a variety of different tissues, including skin, esophagus, trachea, heart, and lung (Keane and Badylak 2014). Creation of biologic scaffolds involves removing the endogenous cell population while maintaining the gross structure of the ECM and its composition (Fig. 8.2). While a variety of methods have been utilized to generate acellular scaffolds, those that minimize destruction to ECM components and loss of mechanical properties are viewed as most desirable, as these are critical inputs to regulating cellular behavior. The most common techniques utilize a series or combination of chemical agents (e.g., detergents, solvents, acids/bases, and hypotonic/hypertonic solutions), biological agents (e.g., enzymes or chelating agents), and physical methods (e.g., freeze/thaw cycles, sonication) and vary in duration based on the species, organ, and method of decellularization [reviewed in (Crapo et al. 2011)] (see Table 8.1 for a list of commonly utilized reagents). In general, most protocols last from 1 to 7 days.
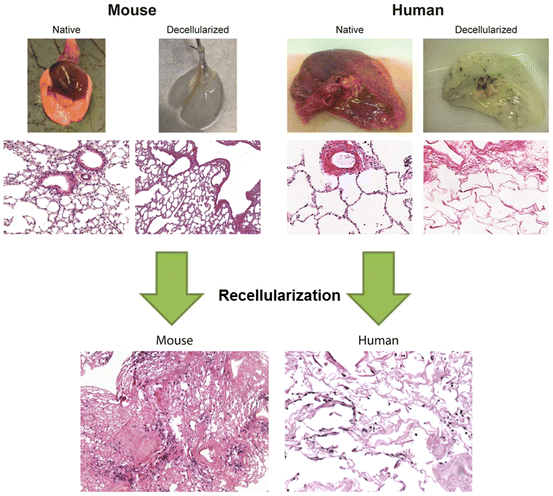
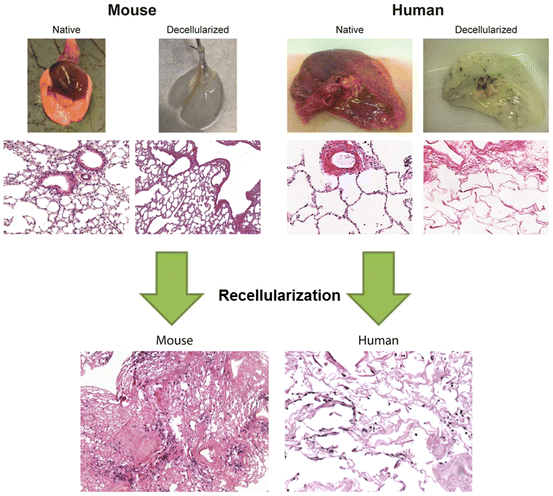
Fig. 8.2
Overview of the decellularization and recellularization process. Representative images of native and decellularized lungs from mice and humans (upper panel) demonstrating loss of pigmentation following decellularization, whereby the lungs become translucent white in color. H&E staining reveals complete cellular removal and gross maintenance of histological architecture. Histological analysis following recellularization with murine alveolar epithelial cells (C10) (left) and human bronchial epithelial cells (HBE) (right) into acellular mouse and human lung slices. Cells can be seen to have attached to the acellular lungs after 1 day of slice culture
Table 8.1
Chemical agents commonly used in whole organ decellularization
Agent | Properties |
---|---|
Triton X-100 | Nonionic detergent used to solubilize proteins; mild non-denaturing detergent |
Sodium deoxycholate (SDC) | Water-soluble ionic detergent used for disrupting and dissociating protein interaction |
Sodium dodecyl sulfate (SDS) | Anionic surfactant used for lysing cells and unraveling proteins |
3-[(3-cholamidopropyl)dimethylammonio]-1-propanesulfonate (CHAPS) | Non-denaturing zwitterionic detergent used to solubilize proteins |
Ethylenediaminetetracetic acid (EDTA) | Chelating agent that binds to calcium and prevents joining of cadherins between cells, preventing clumping of cells grown in liquid suspension, and detaching adherent cells. Can also be used to inhibit metalloproteinases |
Peracetic acid | Removes residual nucleic acids, sterilization agent |
Antibiotics | Typically Penicillin, Streptomycin, and an anti-mycotic Amphotericin |
Other | DNase, RNase, and heparin |
In the lung, maintenance of both large and small airways and vessels, as well as the more delicate alveolar, capillary, and lymphatic systems, is vital. Thus far, several techniques have been reported for decellularization of healthy mouse, rat, porcine, non-human primate, and human lungs (Tables 8.2 and 8.3). Perfusion decellularization has been most commonly utilized for whole organ decellularization, but there have also been reports of excising segments from native lung and decellularizing smaller segments (O’Neill et al. 2013; Nakayama et al. 2013; Zhou et al. 2014; Parker et al. 2014). Detergents are most commonly utilized for perfusion-based lung decellularization and several works have compared differences in the proteomic composition and mechanical properties of the final acellular scaffold and potency for recellularization in the different detergent-based protocols (Wallis et al. 2012; Gilpin et al. 2014; O’Neill et al. 2013). The most commonly utilized detergents for lung are either the ionic detergents sodium deoxycholate (SDC) or sodium dodecyl sulfate (SDS) in combination with the nonionic detergent Triton X-100 (Bonenfant et al. 2013; Bonvillain et al. 2012; Booth et al. 2012; Daly et al. 2012a; Jensen et al. 2012; Longmire et al. 2012; Sokocevic et al. 2013; Wagner et al. 2014a, b; Wallis et al. 2012; Gilpin et al. 2014; Ott et al. 2010; Song et al. 2011; Parker et al. 2014). Alternatively, zwitterionic detergents such as 3-[(3-cholamidopropyl)dimethylammonio]-1-propanesulfonate (CHAPS) have also been used (Petersen et al. 2010, 2011, 2012; O’Neill et al. 2013). Further, most protocols incorporate hypertonic lysis of cells with sodium chloride (NaCl) as well as a DNase and/or RNase step to clear residual DNA and RNA which is difficult to remove. There is, as yet, no consensus on the best route of administration and removal of decellularization agents (i.e., vascular versus airway perfusion or a combination). While both vascular-only perfusion and a combination of vascular and airway perfusion have produced acellular scaffolds capable of supporting recellularization, how differences in protocols and routes of administration for decellularization reagents might affect recellularization protocols or potential immunogenicity of implanted scaffolds is not yet known.
Table 8.2
Compiled studies of ex vivo lung bioengineering using decellularized whole lung scaffolds
Reference | Scaffold | Study objective | Method of decellularization | Length of decellularization process | Endpoint assessments |
---|---|---|---|---|---|
Kuttan et al. (1981) | Alveolar basement membrane (calf, dog, rabbit, adult/newborn rat) | Basement membrane | Filtered distal lung homogenate, saline, 4 % Triton X-100 with protease inhibitors, NaHCO3 rinse, distilled H2O rinse | 26–52 h depending on homogenate volume | Histology, immunofluorescence, electron microscopy, amino acid analysis, carbohydrate analysis |
Lwebuga-Mukasa et al. (1986) | Acellular alveolar versus amniotic basement membranes | Differentiation on different basement membranes | Distilled H2O, 0.1 % Triton X-100, 2 % SDC, NaCl, pancreatic DNase type 1S | >2 days | Cell attachment and morphology |
Price et al. (2010) | Mouse (female C57/BL6) acellular lungs | Effect of matrix on spatial engraftment of E17 fetal lung homogenate | Airway and vascular perfusion: distilled H2O, 0.1 % Triton X-100, SDC, NaCl, porcine pancreatic DNase | 3 days (approximately 63 h) | Histology, quantification of ECM proteins, immunofluorescence, SEM, function with flexivent, bioreactor with fetal type II cells |
Petersen et al. (2010) | Rat acellular lungs (male Fischer 344) | Development of bioartificial lung for orthotopic transplantation | Vascular perfusion only (1–5 mL/min with less than 20 mmHg arterial pressure) CHAPS, NaCl, EDTA, PBS | 4 h | Histology, immunofluorescence, DNA quantification assay, collagen assay, GAG assay, western blots, SEM, TEM, micro-CT imaging |
Cortiella et al. (2010) | Rat acellular lung (Sprague Dawley) | Comparison of matrices including decellularized rat lung in ability to support mESCs | Fast freeze/thaw cycles, 1 % SDS, DNase, RNase, PBS, Penicillin/Streptomycin, Amphotericin, DMEM | >6 weeks | Quantification of DNA, immunohistochemistry, confocal microscopy, flow cytometry, 2 photon microscopy, presence of SPA |
Ott et al. (2010) | Rat acellular lung (Sprague Dawley) | Development of bioartificial lung for orthotopic transplantation | Vascular perfusion only: pulmonary artery pressure kept constant At 80 cmH2O, heparinized PBS with 0.1 % SDS, deionized water, Triton X-100, and PBS with Penicillin, Streptomycin, Amphotericin B | 3 days (approximately 75 h) including incubation with antibiotics | Histology, morphology, mechanical function, fluoroscopy, gas exchange, transplantation, protein analysis |
Song et al. (2011) | Rat acellular lung (Sprague Dawley) | Orthotopic transplantation | Vascular perfusion only: pulmonary artery pressure kept constant at 80 cmH2O, heparinized PBS with 0.1 % SDS, deionized water, Triton X-100, and PBS with Penicillin, Streptomycin, Amphotericin B | 3 days (approximately 75 h) including incubation with antibiotics | Histology, immunohistochemistry, morphology, fluoroscopy, functional analysis, transplantation seeded lungs with fetal pulmonary cells and pulmonary artery and vein with endothelial cells |
Shamis et al. (2011) | Rat acellular liver and lung (Lewis) | Cellular differentiation on 3D in vitro scaffold | Lung lobes cut into 300 μm thick, 0.5 % Triton X-100, 10 mm ammonia, mechanical disruption, PBS, distilled water | N/A | Histology, TEM, environmental scanning, PCR, immunohistochemistry, liquid chromatography with tandem mass spectrometry |
Daly et al. (2012a) | Mouse acellular lung (C57BL/6; BALB/C) | Initial binding and recellularization of MSCs in acellular scaffold; directed seeding with integrin blocking | Airway and vascular perfusion: distilled H2O, 0.1 % Triton X-100, 2 % SDC, NaCl, pancreatic DNase type 1S | 3 days (approximately 72 h) | Histology, immunofluorescence, EM, perfusion to assess vascular continuity, mass spectrometry, western blot, lung mechanics with flexivent, innoculation of bone marrow-derived MSCs |
Wallis et al. (2012) | Mouse acellular lung and lung slices (BALB/C) | Comparison of detergent-based decellularization protocols | Airway and vascular perfusion. Three different protocols tested: (1) H2O, 0.1 % Triton X-100, 2 % SDC, NaCl, porcine pancreatic DNase; (2) PBS, 0.1 %SDS, 0.1 %Triton X-100; (3) PBS, CHAPS, NaCl, EDTA, DNase, FBS | 3 days (approximately 72 h) | Immunohistochemistry, mass spectrometry, western, mechanical analysis, gelatinase, DNase, RNase, comparative recellularization with MSCs and C10s |
Bonvillain et al. (2012) | Normal rhesus macaque acellular lung | Initial binding and recellularization of MSCs in acellular scaffold | Airway and vascular perfusion: PBS, EDTA, Penicillin/Streptomycin at initial harvest: pulmonary artery: PBS + heparin + sodium nitroprusside with pressures 25–30 mmHg; then trachea and vasculature: deionized H2O, 0.1 % Triton X-100, 2 %SDC, NaCl, bovine pancreatic DNase | 2–3 days (approximately 48–72 h) | Histology, morphology, immunohistochemistry, western blot, genomic DNA, proteomics, seeding with bone marrow and adipose-derived rhesus MSCs |
Longmire et al. (2012) | Mouse acellular lung and lung slices (C57/BL6) | Seeding with and differentiation of mESCs-derived endodermal lung precursors | Airway and vascular perfusion: distilled H2O, 0.1 % Triton X-100, 2 % SDC, NaCl, pancreatic DNase type 1S | 3 days (approximately 72 h) | Evaluation of ability to differentiate mESCs into lung precursor cells |
Jensen et al. (2012) | Mouse acellular lung (C57BL/6) | Comparison of timing of decellularization, coating of decellularized matrices, and support of mESCs differentiated into alveolar epithelial cells | Airway and vascular perfusion: 0.1 % Triton X-100, 2 % SDC, NaCl, porcine pancreatic DNase, PBS | 1 vs. 3 days (approximately 24 h vs. 50 h) | Histology, morphology, EM, western blot, gelatinase assay, immunofluorescence, mechanical properties with flexivent, support of differentiated mESCs within scaffold, subcutaneous implantation of scaffold |
Petersen et al. (2012) | Rat acellular lung (Fisher 344) | Comparison of different detergent-based decellularization protocols | Two approaches: (1) vascular perfusion CHAPS, NaCl, EDTA, PBS; (2) NaCl, EDTA, SDS | 4 h | Histology, collagen assay, elastin assay, GAG assay, DNA assay, mechanical testing with linear strips |
Mishra et al. (2012) | Rat acellular lung | Creation of perfusable human lung cancer nodules | Vascular perfusion only: pulmonary artery pressure kept constant at 80 cmH2O, heparinized PBS with 0.1 % SDS, deionized water, Triton X-100, and PBS with Penicillin, Streptomycin, Amphotericin B | 3 days (approximately 72 h) including incubation with antibiotics | Recellularization with human A549, H460, or H1299 and cultured with perfused, oxygenated media for 7–14 days |
Bonenfant et al. (2013) | Mouse acellular lung and lung slices (C57BL/6) | Effect of time to necropsy, length of storage, and two different methods of sterilization of construct | Airway and vascular perfusion: distilled H2O, 0.1 % Triton X-100, 2 % SDC, NaCl, pancreatic deoxyribonuclease type 1S, MgSO4, CaCl2, Penicillin, Streptomycin | 3 days (approximately 72 h) | Histology, immunohistochemistry, morphology, mass spectrometry, seeded lungs with MSCs and C10 epithelial cell line |
Sokocevic et al. (2013) | Mouse acellular lung and lung slices (C57BL/6) | Effect of recipient age and elastase, or bleomycin injury on decellularization and recellularization | Airway and vascular perfusion: distilled H2O, 0.1 % Triton X-100, 2 % SDC, NaCl, pancreatic deoxyribonuclease type 1S, MgSO4, CaCl2, Penicillin, Streptomycin | 3 days (approximately 72 h) | Histology, immunohistochemistry, mass spectrometry, inoculation with MSCs and C10 cells |
Sun et al. (2014) | Rat and mouse acellular lung slices | Engraftment and survival of fibroblasts through a β1-integrin and FAK-dependent pathway through ERK | CHAPS, NaCl, EDTA | 1 day | Histology, SEM, immunohistochemistry, DNA assay, recellularization with mouse A9 cells |
Table 8.3
Summary of decellularization methods for human and porcine lungs
Reference | Species | Decellularization agents | Perfusion parameters | Instillation route | Days |
---|---|---|---|---|---|
Petersen et al. (2010) | Human | CHAPS, NaCl, and EDTA | Constant pressure (25 mmHg) | Airway and vasculature | 1 |
Booth et al. (2012) | Human/IPF | Triton X-100, SDC, NaCl, DNase | Unspecified | Airway and vasculature | 3 |
Gilpin et al. (2014) | Human/porcine | SDS, Triton X-100 | Constant pressure (30 cmH2O) | Vascular | 4–7 |
O’Neill et al. (2013) | Human/porcine | (a) SDS or (b) CHAPS or (c) Tween-20, SDC, peracetic acid | None—lung segments and agitation | N/A | 1 |
Nichols et al. (2013) | Human/porcine | Freeze/thaw; graded SDS perfusion | Varying flow rates (100–500 mL/h) | Airway and vasculature | 7 |
Price et al. (2015) | Porcine | Triton X-100, SDC, NaCl | 12–25 mL/min (15 mmHg) | Airway and vasculature | 1 |
Parker et al. (2014) | Human/IPF | SDS, Triton, NaCl | None—thin lung slices | N/A | 2 |
Wagner et al. (2014b) | Human/porcine | Triton X-100, SDC, NaCl, DNase peracetic acid | Constant flow rates (1 L, 2 L, 3 L/min) | Airway and vasculature | 3 |
Wagner et al. (2014a) | Human/COPD | Triton X-100, SDC, NaCl, DNase peracetic acid | Constant flow rate 2 L/min | Airway and vasculature | 3 |
While standards do not yet exist for generating decellularized scaffolds, Crapo et al. proposed three minimal criteria: (1) <50 ng dsDNA per 1 mg ECM dry weight; (2) <200 bp DNA fragment length; (3) absence of visible nuclear content in histological sections by 4′,6-diamidino-2-phenylindole (DAPI) or hematoxylin–eosin (H&E) staining (Crapo et al. 2011). However, these criteria do not set forth additional characteristics which acellular scaffolds should have, such as preservation of specific ECM components, mechanical properties, or biological activity.
8.2.2 Scaling Up Decellularization Protocols for the Clinic
Scaling up decellularization protocols from rodent models to potential large animal xenogeneic sources (e.g., porcine) or human scaffolds presents additional challenges. In addition to anatomical differences, the larger size is a significant consideration for the actual decellularization technique. While rodent and macaque lungs can be decellularized by hand, higher pressures and volumes must be utilized for sufficient inflation of perfusion pathways (e.g., vasculature, airways, etc.) in larger organs to ensure that perfused solutions and the ensuing cellular debris are cleared from the lungs. All published protocols to date for decellularizing whole large animal or human lungs utilize perfusion pumps to generate acellular scaffolds which can support recellularization (Wagner et al. 2014a, b; Nichols et al. 2013; Booth et al. 2012; Petersen et al. 2010; Price et al. 2015; Gilpin et al. 2014). While not clinically translational for transplantation, human and porcine lung segments have also been decellularized using small segments (Zhou et al. 2014; O’Neill et al. 2013; Nakayama et al. 2013; Parker et al. 2014) (Table 8.3).
A variety of post-decellularization techniques can be utilized to assess differences in potential decellularization techniques, including histologic, immunofluorescent staining, and DNA detection and quantification (Fig. 8.2). Further, proteomic analysis is a powerful tool which can be used to help delineate differences between protein loss and retention in protocols and can aid in the selection of optimal protocol parameters such as flow rates or pressures (Wagner et al. 2014b). For example, proteomic analysis can help delineate the impact of different steps and parameters in decellularization protocols (e.g., flow rate, pressure, rinse volumes, etc.) which preferentially retain certain ECM components or minimize retention of cellular-associated proteins (Wagner et al. 2014a, b; Booth et al. 2012).
8.2.3 Residual ECM and Other Proteins
The lung ECM itself has long been known to provide instructional cues during prenatal development as well as postnatally in maintaining tissue homeostasis and directing remodeling responses after injury (Fernandes et al. 2010; Lin et al. 2010; Mariani et al. 1997; Nguyen and Senior 2006; Rippon et al. 2006). While synthetic scaffolds offer the advantages of precise control and the ability to be more readily mass produced and stored, synthetic materials lack the biologically inductive capabilities observed in acellular scaffolds. Acellular scaffolds retain native integrin-binding sites in their correct spatial arrangement, in addition to preserving organ macro-architecture. A number of studies have shown their ability to induce phenotypic differentiation in the absence of the use of additional growth factors. While synthetic materials could be engineered to include specific integrin-binding sites to enhance cell adhesion [e.g., Arg-Gly-Asp (RGD) binding sites], it remains unknown what specific integrin-binding sites need to be included and in what spatial arrangement they need to be. Hybrid materials, consisting of synthetic and acellular matrix components, are also an attractive possibility and could be utilized to enhance cell adhesion and biological activity while taking advantage of the ability to more precisely manufacture scaffolds or scaffold components with synthetic materials (Ingenito et al. 2010, 2012; Cortiella et al. 2010; Nichols et al. 2013; Fischer et al. 2011). (Differences between acellular and synthetic scaffold approaches summarized in Table 8.4.)
Table 8.4
Comparison of biologic versus synthetic scaffold approach for ex vivo bioengineering
Biologic (acellular) scaffold | Synthetic scaffold | |||
---|---|---|---|---|
Differentiation and engraftment cues | + | Retains native integrin-binding sites | − | Lacks specific integrin-binding sites (must be engineered into scaffolds) |
Immunogenicity | + | Antigen removal during decellularization | +/− | Unknown/variable depending on material |
Manufacturability | + | Native architecture largely retained | − | Complex architecture |
− | Large variability between donor scaffolds | + | Precise control possible (i.e., repeatability) | |
Long-term storage | − | Degradation with long-term storage | + | Improved storage stability |
Owing to the importance of ECM components, retention of key ECM components is a critical parameter to control and assess as an endpoint when evaluating potential decellularization protocols. The precise combination of ECM proteins that must be retained to preserve the ability of the acellular scaffold to give organotypic cues for cellular differentiation and functional tissue level assembly remains unknown. The major structural and functional molecules in the ECM include both proteins such as collagens, elastin, fibronectin, and laminins as well as a variety of glycoproteins including the glycosaminoglycans (GAGs). Collagens are important structural components of the lung and are responsible for overall mechanical strength, while elastin gives the lung its elastic properties of reversible distension and intrinsic recoil. GAGs help control macromolecular and cellular movement across the basal lamina and may play a role in the mechanical integrity of the lung. Matrix molecules are generally highly conserved proteins in eukaryotic organisms. This may theoretically explain the lack of an adverse immune response seen in xenotransplantation of other decellularized organs such as skin, trachea, and esophagus (Jungebluth et al. 2012a, b; Keane and Badylak 2014; Fishman et al. 2013).
There are a variety of techniques which have been used to evaluate ECM components, including histology, immunohistochemistry, Western Blotting, mass spectrometry-based proteomics, and component-specific assays such as Picrosirius, Fastin elastase, etc. (Table 8.2). The majority of decellularization techniques used for lung results in loss of elastin and sulfated GAGs in all species (Ott et al. 2010; Petersen et al. 2010; Price et al. 2010; Bonenfant et al. 2013; Bonvillain et al. 2012; Daly et al. 2012a; Jensen et al. 2012; Sokocevic et al. 2013; Wallis et al. 2012). In head to head comparison studies in perfused lungs, SDS and SDC have been found to retain more elastin as compared to CHAPS-based protocols (Petersen et al. 2012; Wallis et al. 2012). However, despite the differences in retention of ECM components, inoculated cells appear to behave similar in the repopulation assays currently used (including histological and immunofluorescence evaluation). Therefore, it remains unknown if there is an optimal decellularization protocol, and if so, which is best suited for translation to the clinic.
In contrast to traditional protein detection methods, mass spectrometry-based proteomics is gaining traction as an invaluable tool for assessing acellular scaffolds. In addition to detecting ECM composition and residual proteins in acellular scaffolds, it has been used for distinguishing differences between decellularization methods or lung origin, including disease states or donor age (Wallis et al. 2012; Bonvillain et al. 2012; Sokocevic et al. 2013; Wagner et al. 2014a, b; Booth et al. 2012; Nakayama et al. 2013; Gilpin et al. 2014). One particularly striking and consistent result among the various groups utilizing this analysis approach is the amount and breadth of non-ECM proteins detected in the scaffold following decellularization. In particular, cytoskeletal elements and histones appear to be retained in the scaffolds, while lesser secreted proteins are detected. This suggests that transmembrane proteins and their associated cytoskeletal elements may remain anchored to the ECM with the currently used decellularization protocols. The impact of these residual proteins on recellularization, including potential immunogenicity, remains unknown.
8.2.4 Mechanical Assessments of Decellularized Scaffolds
A variety of in vitro assessments have been utilized to begin to establish metrics for assessing the potential functionality of acellular scaffolds. Investigators have explored both micro- (Melo et al. 2014a, b; Nonaka et al. 2014; Booth et al. 2012) and macro-scale (Daly et al. 2012a; Wallis et al. 2012; Nichols et al. 2013) mechanical measurements of acellular lungs as well as force tension relationships in linear strips of decellularized lungs (Petersen et al. 2012; O’Neill et al. 2013). While techniques such as atomic force microscopy (AFM) are useful in obtaining topographical information and initially assessing mechanical properties of the scaffolds (Melo et al. 2014a, b; Nonaka et al. 2014; Booth et al. 2012), these results do not necessarily translate to recellularization and functional performance. Traditional lung mechanics testing of acellular scaffolds has shown that in the absence of cells and surfactants, acellular scaffolds are stiffer than their naïve counterparts (Daly et al. 2012a). Introduction of exogenous surfactant into the acellular scaffolds can partially restore lung compliance (Daly et al. 2012a). This is an important finding and indicates that during recellularization strategies, serial measurements of lung mechanics could be used as a non-invasive and non-destructive means to assess functionality of the regenerating scaffold. For example, decreases in elastance could be used as a measurement of de novo surfactant production.
8.3 Recellularization
8.3.1 Recellularization of Acellular Scaffolds for Bioengineering New Lung
The lung is comprised of many different cell subtypes and all uniquely contribute to some critical aspect of lung function (Morrisey and Hogan 2010). This heterogeneous cell population is replenished by resident stem or progenitor cells following injury. Regeneration of lungs suitable for transplantation will require some minimal restoration of these subtypes for subsequent long-term functionality. While a variety of cell sources are being investigated for recellularizing acellular scaffolds, obtaining sufficient cell numbers with any source remains a significant open question. The ideal solution is to use an autologously derived source of cells to minimize post-transplantation immune complications, which are a significant cause of morbidity in transplanted patients. One potential source is the use of fully differentiated primary adult cells, which ideally would come from an autologous source. However, using this approach, the multiple cell types would need to be isolated from the eventual transplant recipient, grown to sufficient numbers ex vivo and then further be able to be used in a recellularization approach to restore functionality. While it has been shown that a strategy such as integrin blocking can be used to direct initial cell engraftment of a single cell population (Daly et al. 2012a; Sun et al. 2014), scaling this clinically and further adding the complex challenge of uniquely directing the right cell population to a specific architectural location would be challenging. Alternatively, autologous endogenous lung progenitor cells from the various compartments could be utilized (e.g., distal and proximal epithelial progenitor cells, endothelial progenitor cells, etc.) along with stromal cells to recellularize acellular scaffolds. However, the same challenges of obtaining sufficient cell numbers for an initial seeding strategy and directing cells to their correct compartment remain. In both instances, however, it remains unknown if normal cells could be obtained from a patient with a preexisting lung disease or if isolated diseased cells could be gene-corrected prior to subsequent recellularization. However, recent work indicates that the scaffold may more significantly contribute to phenotype than cell origin (Parker et al. 2014). An allogeneic cell source could also be used, but this re-introduces the potential for immune complications following transplantation. Furthermore, the identification of bona fide lung progenitor cells in the adult human lung remains controversial.
A potentially more appealing autologous approach is the use of induced pluripotent stem (iPS) cells, which are derived from reprogramming somatic cells to a stem-cell-like state. While iPS cells avoid the ethical controversies surrounding the use of embryonic stem cells (ESCs)—stem cells derived from the inner blastocyst of in vitro fertilized embryos—they have been shown to retain epigenetic memory of their tissue origin and have been shown to form teratomas. iPS cells are typically derived from dermal fibroblasts, and thus, differentiating them into the various lung cell types has been challenging. However, despite this limitation, recent work has demonstrated that human iPS cells can be differentiated into cells expressing a distal pulmonary epithelial cell phenotype and seeded into acellular human lung scaffolds (Ghaedi et al. 2013; Huang et al. 2014). These results further encourage the use of this approach in moving towards the clinic.
Other potential approaches include the use of fetal homogenates or ESCs. As previously mentioned, ethical concerns remain for either of these approaches, as well as the potential for teratoma formation with ESCs. While initial studies have shown that ESCs can engraft in acellular murine lungs (Longmire et al. 2012; Cortiella et al. 2010), seeding into acellular lungs was not sufficient to induce differentiation and thus optimized in vitro differentiation protocols must be used in conjunction with seeding and repopulation strategies. ESC-derived murine Nkx2-1GFP+ progenitor cells were able to recellularize acellular murine lungs and form alveolar structures, while in contrast, seeding with undifferentiated ESCs resulted in nonspecific cell masses in distal regions of acellular lungs. Fetal homogenates have the distinct advantage of containing all necessary cell populations which have been shown to have some capacity for self-assembly. Further, these cells have been successfully used in the current rodent models of ex vivo regeneration and transplantation. However, in both instances, ethical concerns remain in obtaining these cells and the use of immunosuppressive drugs post-transplantation remains unknown. Tables 8.5 and 8.6 are a summary of recellularization approaches in animal and human models and the phenotypic adoption of seeded cells.
Table 8.5
Distribution and phenotype of cells seeded onto animal models of acellular scaffolds
Reference | Cells used for seeding | Scaffold | Route | Duration (days) | Distribution | Final phenotype |
---|---|---|---|---|---|---|
Lwebuga-Mukasa et al. (1986) | AECII | Acellular alveolar vs. amniotic basement membranes | Direct seeding | 8 | N/A | Alveolar matrices: ATI; amnionic membranes ATII |
Cortiella et al. (2010) | mESC | Rat (Sprague Dawley) acellular lung | Trachea | 21 | Proximal-distal regional specific CC10, proSP C expression) | Tracheo-bronchial: CC10, Ck18; distal lung: proSPC, CD31, Pdgfrα |
Ott et al. (2010) | HUVEC (DsRed) | Rat acellular lung | Pulmonary artery | 9 | All vessels | Endothelial cells |
A549 | Rat acellular lung | Trachea | 9 | Airways/alveoli | Airway/alveolar epithelium | |
HUVEC (DsRed) | Rat acellular lung | Pulmonary artery | 9 | Entire vasculature | Endothelial cells | |
Rat fetal lung cells (GD19-20) | Rat acellular lung | Trachea | 9 | Airways/alveoli | proSp-a, proSPC, Ttf-1/Nkx2.1 (AECII); T1α (AECI); vimentin (fibroblast) | |
Petersen et al. (2010) | Neonatal (7d) lung epithelial cells (rat) | Rat acellular lungs (Fischer 344) | Trachea | 8 | Alveolar, small airways | CCSP (Club cell), proSPC (ATII), Aqp5 (ATI), Ck14 (basal cell) |
Lung vascular endothelium (rat) | Rat acellular lungs (Fischer 344) | Pulmonary artery | 7 | Microvascular | CD31 | |
Price et al. (2010) | Fetal lung (E17) | Ms acellular lungs | Tracheal | 7 | Alveolar | CK18+/proSPC+ (ATII); CD11b, Aqp5, CCSP, CD31, and vimentin |
Daly et al. (2012a) | mBM-MSCs | Ms acellular lung | Trachea | 28 | Parenchymal > airway (squamous) | MSCs: no evidence for transdifferentiation |
C10-hAECII (non-tumorigenic) | Ms acellular lung | Trachea | 28 | Parenchymal | N/A | |
Ott et al. (2010) | Rat fetal (GD17-20) pneumocytes | Rat acellular lung | Trachea | 14 | Alveolar/distal bronchioles > trachea/bronchi | CCSP (airways); Ttf-1, proSPC (alveolar) |
HUVEC | Athymic nude rat | Pulmonary artery | 14 | Proximal to distal vasculature | CD31pos | |
Shamis et al. (2011) | Ms AECII (primary or P2) | Acellular lung microscaffold | Direct seeding | 22 | Alveolar | proSPC/SPC (ATII-like, from primary or cultured ATII); Aqp5, PDPN (ATI); CCSP (primary) |
Wallis et al. (2012) | mBM-MSCs | Ms acellular lung | Trachea | 14 | Alveolar | MSCs |
C10-hAECII (non-tumorigenic)
![]() Stay updated, free articles. Join our Telegram channel![]() Full access? Get Clinical Tree![]() ![]() ![]() |