3 Wheezes are high-pitched, continuous sounds generated by airflow through narrowed airways. Causes of such narrowing include airway smooth muscle constriction, edema, secretions, intraluminal obstruction, and collapse because of poorly supported walls. These individual pathophysiologic features are discussed in Chapters 4 through 7. For reasons that are also described later, the diameter of intrathoracic airways is less during expiration than inspiration, and wheezing generally is more pronounced or exclusively heard in expiration. However, because sufficient airflow is necessary to generate a wheeze, wheezing may no longer be heard if airway narrowing is severe. In conditions such as asthma and chronic obstructive pulmonary disease, wheezes originate in multiple narrowed airways and are generally polyphonic, meaning they are a combination of different musical pitches that start and stop at different times during the expiratory cycle. In contrast, wheezing sounds tend to be monophonic when they result from focal narrowing of the trachea or large bronchi. When the site of narrowing is the extrathoracic airway (e.g., in the larynx or the extrathoracic portion of the trachea), the term stridor is used to describe the inspiratory wheezing-like sound that results from such narrowing. Physiologic factors that relate the site of narrowing and the phase of the respiratory cycle most affected are described later in this chapter and shown in Figures 3-20 and 3-21. Table 3-1 summarizes some of the pulmonary findings commonly seen in selected disorders affecting the respiratory system. Many of these are mentioned again in subsequent chapters when the specific disorders are discussed in more detail. Table 3-1 TYPICAL CHEST EXAMINATION FINDINGS IN SELECTED CLINICAL CONDITIONS *May be altered by collapse of underlying lung, which will increase transmission of sound. Clubbing is a change in the normal configuration of the nails and the distal phalanx of the fingers or toes (Fig. 3-1). Several features may be seen: (1) loss of the normal angle between the nail and the skin, (2) increased curvature of the nail, (3) increased sponginess of the tissue below the proximal part of the nail, and (4) flaring or widening of the terminal phalanx. Although several nonpulmonary disorders can result in clubbing (e.g., congenital heart disease with right-to-left shunting, endocarditis, chronic liver disease, inflammatory bowel disease), the most common causes clearly are pulmonary. Occasionally, clubbing is familial and of no clinical significance. Carcinoma of the lung (or mesothelioma of the pleura) is the single leading etiologic factor. Other pulmonary causes include chronic intrathoracic infection with suppuration (e.g., bronchiectasis, lung abscess, empyema) and some types of interstitial lung disease. Uncomplicated chronic obstructive lung disease is not associated with clubbing, so the presence of clubbing in this setting should suggest coexisting malignancy or suppurative disease. Chest radiographs usually are taken in two standard views—posteroanterior (PA) and lateral (Fig. 3-2). For a PA film, the x-ray beam goes from the back to the front of the patient, and the patient’s anterior chest is adjacent to the film. The lateral view is taken with the patient’s side against the film, and the beam is directed through the patient to the film. If a film cannot be taken with the patient standing and the chest adjacent to the film, as in the case of a bedridden patient, then an anteroposterior view is taken. For this view, which is generally obtained using a portable chest radiograph machine in the patient’s hospital room, the film is placed behind the patient (generally between the patient’s back and the bed), and the beam is directed through the patient from front to back. Lateral decubitus views, either right or left, are obtained with the patient in a side-lying position, with the beam directed horizontally. Decubitus views are particularly useful for detecting free-flowing fluid within the pleural space and therefore are often used when a pleural effusion is suspected. Figure 3-2 Normal chest radiograph. A, Posteroanterior view. B, Lateral view. Compare with Figure 3-3 for position of each lobe. Knowledge of radiographic anatomy is fundamental for interpretation of consolidation or collapse (atelectasis) and for localization of other abnormalities on the chest film. Lobar anatomy and the locations of fissures separating the lobes are shown in Figure 3-3. Localization of an abnormality often requires information from both the PA and lateral views, both of which should be taken and interpreted when an abnormality is being evaluated. As can be seen in Figure 3-3, the major fissure separating the upper (and middle) lobes from the lower lobe runs obliquely through the chest. Thus it is easy to be misled about location on the basis of the PA film alone; a lower lobe lesion may appear in the upper part of the chest, whereas an upper lobe lesion may appear much lower in position. When a lobe becomes filled with fluid or inflammatory exudate, as in pneumonia, it contains water rather than air density and therefore is easily delineated on the chest radiograph. With pure consolidation the lobe does not lose volume, so it occupies its usual position and retains its usual size. An example of lobar consolidation on PA and lateral radiographs is shown in Figure 3-4. Figure 3-4 Posteroanterior (A) and lateral (B) chest radiographs of patient with left upper lobe consolidation due to pneumonia. Anatomic boundary is best appreciated on lateral view, where it is easily seen that normally positioned major fissure defines lower border of consolidation (compare with Figure 3-3). Part of left upper lobe is spared. (Courtesy Dr. T. Scott Johnson.) In contrast, when a lobe has airless alveoli and collapses, it not only becomes more dense but also has features of volume loss characteristic for each individual lobe. Such features of volume loss include change in position of a fissure or the indirect signs of displacement of the hilum, diaphragm, trachea, or mediastinum in the direction of the volume loss (Fig. 3-5). A common mechanism of atelectasis is occlusion of the airway leading to the collapsed region of lung, caused, for example, by a tumor, aspirated foreign body, or mucous plug. All the aforementioned examples reflect either pure consolidation or pure collapse. In practice, however, a combination of these processes often occurs, leading to consolidation accompanied by partial volume loss. An interstitial pattern generally is described as reticular or reticulonodular, consisting of an interlacing network of linear and small nodular densities. In contrast, an alveolar pattern appears fluffier, and the outlines of air-filled bronchi coursing through the alveolar densities are often seen. This latter finding is called an air bronchogram and is due to air in the bronchi being surrounded and outlined by alveoli that are filled with fluid. This finding does not occur with a purely interstitial pattern. Examples of chest radiographs that show diffuse abnormality as a result of interstitial disease and alveolar filling are shown in Figures 3-6 and 3-7, respectively. Compared with the plain chest radiograph, CT of the chest provides greater anatomic detail but is more expensive and exposes patients to a significantly higher dose of radiation. With this technique, a narrow beam of x-rays is passed through the patient and sensed by a rotating detector on the other side of the patient. The beam is partially absorbed within the patient, depending on the density of the intervening tissues. Computerized analysis of the information received by the detector allows a series of cross-sectional images to be constructed (Fig. 3-8). Use of different “windows” allows different displays of the collected data, depending on the densities of the structures of interest. With the technique of helical (spiral) CT scanning, the entire chest is scanned continuously (typically during a single breathhold and using multiple detectors) as the patient’s body is moved through the CT apparatus (the gantry). Chest CT is used extensively in evaluating pulmonary nodules and the mediastinum. It is also quite valuable in characterizing chest wall and pleural disease. As the technology has advanced, CT has become progressively more useful in the diagnostic evaluation of various diseases affecting the pulmonary parenchyma and the airways. With high-resolution CT, the thickness of individual cross-sectional images is reduced to 1 to 2 mm instead of the traditional 5 to 10 mm. As a result, exceptionally fine detail can be seen, allowing earlier recognition of subtle disease and better characterization of specific disease patterns (Fig. 3-9). MRI has several important features in the evaluation of intrathoracic disease. First, flowing blood produces a “signal void” and appears black, so blood vessels can be readily distinguished from nonvascular structures without the need to use intravenous contrast agents. Second, images can be constructed in any plane so that the information obtained can be displayed as sagittal, coronal, or transverse (cross-sectional) views. Third, differences can be seen between normal and diseased tissues that are adjacent to each other, even when they are of the same density and therefore cannot be distinguished by routine radiography or CT. Some of these features are illustrated in Figure 3-10.
Evaluation of the Patient with Pulmonary Disease
Evaluation on a Macroscopic Level
Chest Radiography
Computed Tomography
Magnetic Resonance Imaging
Stay updated, free articles. Join our Telegram channel
Full access? Get Clinical Tree
Evaluation of the Patient with Pulmonary Disease
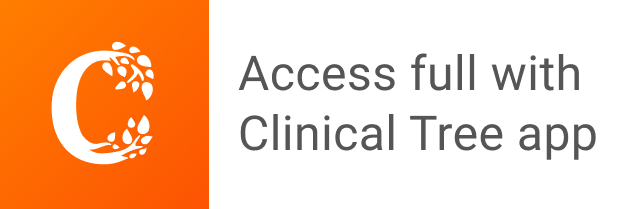