INTRODUCTION
Normal diastolic function allows a ventricle to fill to an adequate volume while maintaining normal diastolic pressures at rest, during exercise, and through a wide range of heart rates. This occurs through the interaction of multiple intracardiac and extracardiac factors (which have been discussed in detail in previous chapters). Failure of these factors to properly operate results in the elevation of left ventricular (LV) diastolic (or filling) pressures at rest or with exertion and consequently the occurrence of cardiac dyspnea, a common denominator in all forms of heart failure regardless of the resting systolic function. However, there are as many pulmonary causes of dyspnea as there are cardiac, and even in patients with known heart disease, the dyspnea may be of a pulmonary origin. Likewise, elevations of right ventricular (RV) filling pressures and right atrial pressures (RAPs) result in right-sided failure and edema as its common manifestation; as with dyspnea, edema has also a common list of noncardiac etiologies. Thus, over the past 20 years, there has been great interest in the development of noninvasive methods to estimate left- and right-sided filling pressures. In this chapter, we will discuss the estimation of left-sided filling pressures and propose an integrated approach. Assessment of right-sided pressures is discussed in detail in Chapter 14 .
PATHOPHYSIOLOGY
Definition of Left Ventricular Filling Pressure
Several pressure measurements are collectively referred to as filling pressures. They include mean left atrial pressure (LAP), mean pulmonary capillary wedge pressure (PCWP) as a corollary of LAP, and LV end diastolic pressure (LVEDP). In addition, diastolic LV pressure (LVP) prior to atrial contraction has been shown to relate closely with mean LAP. It is important, however, to understand the clinical difference between mean LAP (or PCWP) and LVEDP. Although a high LVEDP is indicative of increased LV stiffness at end diastole, many of these patients have normal diastolic LVP prior to atrial contraction, and consequently a normal mean LAP. Therefore, when evaluating patients with dyspnea, it is preferable to utilize indices that provide an estimate of mean LAP rather than LVEDP.
Two-Dimensional Echocardiography
Echocardiography plays a pivotal role in the evaluation of patients presenting with dyspnea of suspected cardiac origin. It provides an accurate assessment of LV size, regional wall motion, and ejection fraction (EF); allows recognition of patterns of LV hypertrophy and remodeling; and provides an accurate assessment of left atrial (LA) enlargement, an extremely frequent finding in heart failure. The anteroposterior LA dimension has been used for decades to determine LA size, primarily because it was the only measurement available in the era of M-mode echocardiography. However, enlargement of the left atrium does not occur symmetrically, and the anteroposterior dimension consistently underestimates the true LA volume ( Fig. 15-1 ). A more accurate assessment is obtained with two-dimensional echocardiography by deriving the volume of the left atrium from planimetry of the chamber in the apical views, using either single or biplane methods. These volume estimates compare well with measurements obtained with three-dimensional–cine computed tomography and magnetic resonance imaging. All subsequent reference in this chapter to the value of determining LA size for the estimation of filling pressures implies that volumes, rather than dimensions, are used. Using echocardiography, the upper limit of normal LA volume, indexed to body surface area, is 28 ml/m 2 .

Echocardiography is currently the most widely used imaging technique to distinguish patients with systolic heart failure from those with diastolic heart failure; the former is characterized by LV dilatation, eccentric hypertrophy (dilated cavity with normal ratio of radius to wall thickness), and depressed EF, and the latter by absence of LV dilatation, preservation of EF (≥50%), and often concentric hypertrophy or remodeling (see Chapters 2 and 6 ). Concentric LV hypertrophy is defined as increased mass with an increased radius/thickness ratio (R/Th), and concentric remodeling is defined as normal mass with increased R/Th. Another frequent finding in patients with diastolic heart failure is a reduction (<8 mm) in the extent of mitral annular descent, an index of the longitudinal vector of contraction and relaxation.
If the echocardiogram in the evaluation of dyspnea demonstrates normal LV size without hypertrophy, normal EF and regional wall motion, normal annular descent, and normal LA size, the cause of dyspnea is unlikely to be cardiac. The contrary may not always be the case, since patients may present with abnormalities of cardiac structure and function, including depressed EF, and have dyspnea of a noncardiac etiology. Perhaps the most reliable finding is the LA size, since, in the absence of atrial fibrillation or mitral regurgitation/stenosis, it represents a “poor man’s” index of the diastolic load imposed by the left ventricle on the atrium (see Chapters 10 and 18 ). In our experience at the Methodist DeBakey Heart Center over the past year, 95% of 2500 patients with echo-Doppler evidence of elevated filling pressures had an enlarged LA volume (unpublished observation).
Doppler Echocardiography—Transmitral and Pulmonary Vein Velocities
Determinants of Transmitral Velocity
With the advent of pulsed-wave (PW) Doppler echocardiography, recordings of transmitral velocity from the apical window at the tips of the mitral leaflets provided an easy-to-use noninvasive tool for the evaluation of diastolic filling. The peak early velocity (E) and its deceleration time (DT), the atrial (A) velocity, and the E/A ratio became popular indices of diastolic function ( Fig. 15-2 ). E/A ratio is a normalized index that reflects early diastolic filling relative to atrial contraction. Isovolumic relaxation time (IVRT) is the interval between the end of ejection and the onset of mitral inflow. IVRT is obtained by recording velocities from an intermediate position between mitral inflow and LV outflow with either PW or continuous wave (CW) Doppler ( Fig. 15-3 ) (see Chapter 10 ).


The determinants of transmitral velocity and its components have been described in detail elsewhere in this volume. Briefly, transmitral velocity is driven by two major factors: flow and the instantaneous transvalvular pressure gradient (see Fig. 15-2 ). During early diastole, active relaxation and LA pressure are the principal determinants of flow and transvalvular gradients. With normal relaxation, LVP decay during isovolumic relaxation occurs rapidly (thus a shorter time constant of relaxation, or Tau), and early ventricular suction is enhanced. This results in a higher E velocity and a shorter DT and IVRT; the opposite occurs with impaired relaxation (longer Tau): less suction, lower E velocity, and longer DT and IVRT. Likewise, with increasing LAP (and a higher LV-LA crossover pressure), IVRT shortens, the early LA-LV transmitral gradient and E velocity increase, and DT shortens; the opposite occurs with a fall in LA pressure.
The influence of relaxation and LA pressure on transmitral velocity explains the different transmitral patterns described in the literature ( Fig. 15-4 ): the normal healthy pattern seen in young hearts; the “impaired relaxation” pattern, also referred to as stage I diastolic dysfunction ; and both the pseudonormal (stage II) and restrictive (stage III) patterns associated with high filling pressures. As discussed later, the relation of these patterns to LAP occurs best in patients with depressed EF.

Determinants of Pulmonary Vein Velocity
Recordings of pulmonary vein velocity (PVV) by PW Doppler are readily obtained in close to 80% of patients during routine examinations. The determinants of the different components of PVV are described in detail in Chapter 10 . In brief, atrial relaxation combined with the normal descent of the annulus during ventricular systole contributes to a rapid decline in LAP (the x-descent) that drives flow into the atrium. This is depicted in the PVV recording as an antegrade flow velocity (S-wave) ( Fig. 15-5 ). Following mitral valve opening, there is a second antegrade velocity (D-wave) coincident with the transmitral E velocity. During atrial contraction, there is a small retrograde velocity (AR) into the pulmonary vein. When the x-descent is replaced by an elevated v-wave (as seen frequently during decompensated systolic heart failure), the S-wave becomes diminished and D becomes accentuated in concert with the increase in E velocity previously described. An S/D ratio of less than 1 (S/D derived using the integral of the velocities) is an index of elevated mean PCWP that, like the E/A ratio and DT, performs more accurately in patients with depressed LVEF.

CLINICAL ASSESSMENT
Estimation of Filling Pressures
Given that impaired relaxation is present in the vast majority of patients with conditions leading to or associated with heart failure ( Box 15-1 ), the influence of LAP on transmitral velocity depends on how much LAP (and LV stiffness) overcomes the opposite effect of impaired relaxation ( Fig. 15-6 ). This opposite interaction can be seen even in patients with normal hearts, in whom a very low LAP or preload results in a lowering of the E velocity and E/A ratio despite normal relaxation. A subtle difference between stage I diastolic dysfunction and normal relaxation with low preload is that the reduction in early filling induced by impaired relaxation results in a greater residual volume in the left atrium at the time of atrial contraction and a compensatory increase in A velocity that often brings the E/A ratio to less than 0.8. In addition, stage I diastolic dysfunction frequently has a DT of greater than 240 msec.
Increased afterload
Aging
Systemic hypertension
Pathologic secondary left ventricular hypertrophy
Hypertrophic cardiomyopathy
Ischemia
Myocardial diseases
Infiltrative cardiomyopathies

In patients with depressed LVEF (<40%), the presence of a stage I pattern is usually associated with normal (<15 mmHg) resting mean LAPs and a PVV S/D ratio greater than 1. In contrast, the pseudonormal (stage II) and restrictive (stage III) patterns are associated with increasing elevation of filling pressures. The stage III pattern is often associated with a diminished A-wave due to the high afterload imposed on the atrium by the elevated LVEDP. In these patients, an S/D ratio of less than 1 in the PVV also implies elevated mean LAP.
Although patients with stage I diastolic dysfunction are often asymptomatic at rest, they are more prone to develop exercise-induced dyspnea and are at risk for developing heart failure over time, particularly the group with a dilated left atrium. These patients often have auscultatory evidence of a fourth heart sound and may have a prominent A-wave in LVP tracing.
In patients with systolic dysfunction (EF <40%), the presence of stage II and III diastolic dysfunction represents a progression of disease and implies a worsening of LV stiffness and higher LAP. Therefore, a gross estimate of mean LAP or PCWP can be inferred from these patterns: Stage I is associated with pressures less than 15 mmHg; stage II is usually associated with pressures between 15 and 25 mmHg; and stage III is associated with pressures greater than 25 mmHg.
Several equations have been proposed in the literature to derive mean PCWP. They all incorporate multiple measurements from transmitral velocity, and some of them add measurements from the pulmonary veins, LA volume, or both. They all have better accuracy in patients with depressed EF and perform poorly in those with normal systolic function. We have found that the equation
Mean PCWP = 17 + ( 5 × E / A ) – ( 0.11 × IVRT )

When estimating mean PCWP, we have not found additional value from recordings of the PVV in patients with systolic heart failure. In contrast, PVV is useful in estimating the LVEDP. During atrial contraction with an elevated LVEDP, LVP may rise over LA pressure, producing a reverse gradient that shortens the duration of the transmitral A velocity (A MV ) relative to the pulmonary vein retrograde A velocity (AR) ( Fig. 15-8 ). The interval AR–A MV has been found to relate directly with LVEDP; whenever AR–A MV exceeds 20 msec, LVEDP is likely to be higher than 20 mmHg. This finding is fairly reliable as long as the P-R interval is within a normal range, that is, is neither too short nor too long.

The progression from stage I diastolic dysfunction to stage II and III is a “two-way street,” meaning that patients may decompensate into the advanced stages and with proper treatment regress back to stage I (i.e., lower filling pressures). The preceding equation has been shown to track well changes in mean PCWP in response to therapy. Application of the Valsalva maneuver can be used to assess reversibility, since the increased intrathoracic pressure during the maneuver causes a decrease in preload and a shift in transmitral velocity from stage III to stage II or from II to I. Stage IV diastolic dysfunction represents a restrictive pattern that fails to reverse with either Valsalva or heart failure therapy. In patients with systolic heart failure, this pattern appears to be a marker of very high LV stiffness and fibrosis and is associated with poor prognosis. In animal models of systolic heart failure, DT has been shown to relate well with LV chamber stiffness. Likewise, in patients with ischemic cardiomyopathy, we have demonstrated an inverse relation between DT and the amount of LV scarring detected by thallium scintigraphy. Patients with a shorter DT (<150 msec) also had less evidence of viability by dobutamine stress, less recovery of function, more heart failure, and a shorter 12-month survival after coronary revascularization.
Limitations of Transmitral and Pulmonary Vein Velocities
As mentioned, the effect of impaired relaxation is to reduce the E velocity, while that of an elevated LAP is to increase the E velocity; thus, the absolute E velocity recorded is the net summation of these two opposing forces (see Fig. 15-6 ). In patients with a depressed LVEF and dilated ventricles, the high atrial pressure and prominent v-wave predominate, resulting in changes in transmitral velocity and PVV, as previously discussed. By contrast, patients with diastolic dysfunction but normal LV size and EF often have elevated minimal LV diastolic pressure, greater preservation of the x-descent in the LAP, and increased filling pressures without prominence of the v-wave. This can result in a stage I pattern despite the presence of elevated filling pressures. In these instances, the transmitral and the pulmonary vein velocities lose sensitivity for detection of high filling pressures, with the exception of the AR–A MV interval, which is less accurate but can provide an estimate of LVEDP.
Although infrequent, a pseudonormal or restrictive transmitral velocity pattern may be seen in patients with diastolic heart failure and mistakenly interpreted as normal diastolic function. Application of the Valsalva maneuver can help by showing regression to stage I diastolic dysfunction. These patients usually have other clues of diastolic heart failure, such as concentric LV remodeling, LA enlargement, or both, and may also demonstrate a prolonged AR–A mv .
Because of the complex interaction of LV relaxation and LAP, transmitral velocity cannot detect elevated filling pressures in normal hearts subjected to a high volume load, such as in acute mitral regurgitation, and is also very limited in the absence of sinus rhythm.
Combination of Transmitral Velocity with Newer Indices of Left Ventricular Relaxation
As discussed at length elsewhere in this volume, there are currently two noninvasive indices of LV relaxation that have gained considerable clinical interest because they are less affected by changes in LAP or preload. They are the flow propagation velocity, derived with color M-mode Doppler, and the early diastolic mitral annular velocity, derived with tissue Doppler (see Chapters 11 and 12 ). We will discuss them in brief.
Flow Propagation Velocity
Following mitral valve opening and under the influence of relaxation and ventricular suction, a sequence of intracavitary pressure gradients develop from base to apex that promote the propagation of mitral inflow toward the LV cavity. The velocity of this flow propagation can be assessed with color Doppler by placing the M-mode cursor within the center of the mitral inflow and obtaining a color M-mode recording, preferably at a sweep speed of 100 mm/s ( Fig. 15-9 ) (see Chapter 11 ). The recording should contain an edge of uniform color during early filling, the slope of which represents the flow propagation velocity ( Vp ). Shifting the color Doppler zero baseline to an aliasing velocity near 70% of the E velocity has been proposed as a method to obtain a distinct color border that improves reproducibility of this measurement. Vp has been shown to be fairly insensitive to changes in LAP and to relate inversely with Tau. A reduced Vp (<40 cm/s) implies impaired relaxation and can be used to distinguish a pseudonormal mitral inflow pattern from a normal one.

Early Annular Diastolic Velocity
The left ventricle has two major vectors of contraction, circumferential and longitudinal. The longitudinal vectors result in shortening of the LV long axis and descent of the mitral annulus toward the apex. The velocity of this motion can be readily obtained with tissue Doppler by placing the sample volume at a corner of the annulus; the recorded velocities reflect the longitudinal vector of contraction and relaxation of that particular wall at its base ( Fig. 15-10 ). Although one can obtain recordings from the septal, lateral, anterior, and inferior corners of the annulus, in routine clinical practice the septal and lateral corners provide most of the needed information. The early diastolic velocity (referred to in the literature as Ea, Em, or E′) has been shown in experimental animal work and in humans to relate well with invasive indices of relaxation such as Tau, (-)dP/dt, and the lowest diastolic LVP. In addition, Ea declines gradually with aging, concordant with other indices of relaxation, and is inversely affected by increasing afterload. In the normally contracting and relaxing heart, Ea is directly altered by changes in preload. However, this relation virtually disappears in abnormal hearts with impaired relaxation. A reduced Ea (<8 cm/s) implies impaired relaxation (whether caused by LV disease or advanced age) and can be used to distinguish a pseudonormal mitral inflow pattern from a normal one.

Estimation of Filling Pressures
Transmitral E velocity relates directly with LAP and inversely with Tau. This can be expressed mathematically as:
E α LAP / Tau
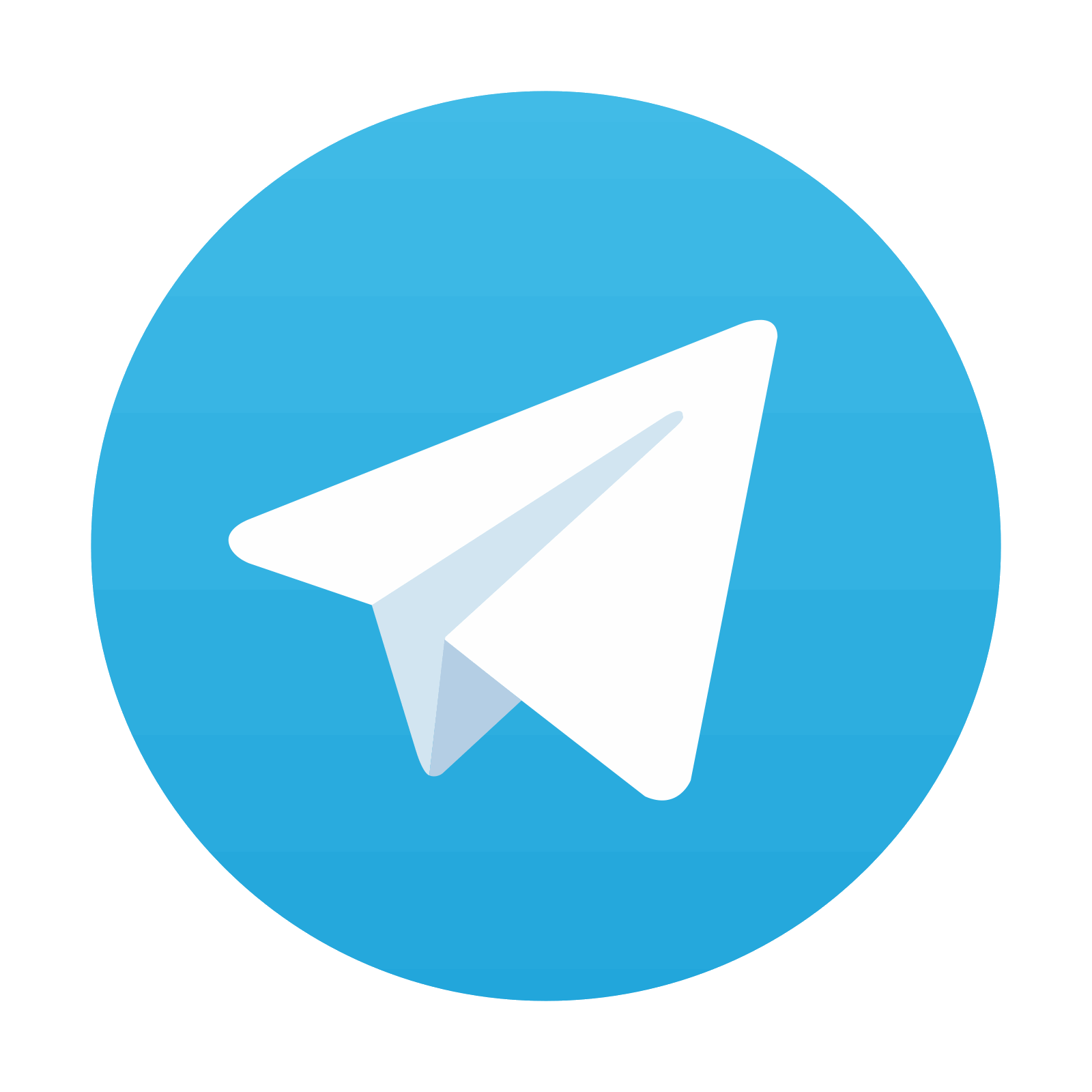
Stay updated, free articles. Join our Telegram channel
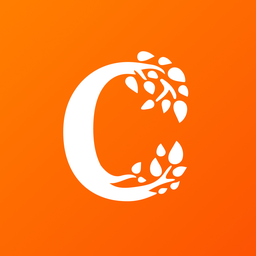
Full access? Get Clinical Tree
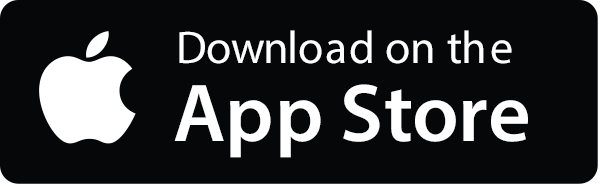
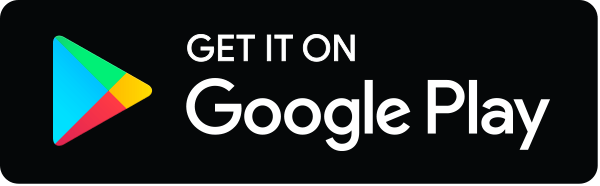