INTRODUCTION
Early Doppler echocardiographic indices of left ventricular (LV) diastolic function have studied the mechanics of left atrial (LA) and ventricular filling. These filling indices have been proven to be useful, providing prognostic information in heart failure patients. However, filling indices have limited accuracy predicting intrinsic parameters of diastolic function due to the con-founding effects of extrinsic loading conditions and valvular hemodynamic performance. Doppler ultrasound and more recently two-dimensional speckle tracking may be applied to study LV myocardial mechanics. Since myocardial mechanical events precede LA and LV filling, they may be potentially less dependent on extrinsic variables and therefore more accurate in characterizing intrinsic myocardial properties.
In this chapter, we will review the role of tissue Doppler and two-dimensional particle tracking–derived myocardial velocities and their derivatives for the assessment of LV diastolic function.
PATHOPHYSIOLOGY
Tissue Doppler Velocities
In technical terms, tissue Doppler echocardiography (TDE) differs from standard Doppler by eliminating the highpass filter and using low gain amplification to display the velocities of the myocardium. TDE velocities may be displayed in spectral pulsed mode or in color-encoded two-dimensional maps superimposed on structural images ( Figs. 12-1 and 12-2 ). The technical principles and limitations of these modalities are similar to those encountered with standard Doppler flow systems. Myocardial velocities may be obtained from multiple locations of the myocardium. In a typical spectral display, the myocardial velocity is a positive waveform representing ventricular systole (S M ) and two waves corresponding to early filling (E M ) and atrial contraction (A M ). From the apical acoustic windows, the diastolic myocardial velocities obtained from any of the LV myocardial segments appear as a mirror image of the mitral inflow early (E) and atrial (A) filling velocities. In normal humans, the peak of E M precedes the peak of LV filling E velocity, suggesting that active relaxation of the myocardium generates negative pressures in the LV cavity that initiate LV filling. TDE velocities are affected not only by regional LV mechanical events, but also by global translation and rotation of the heart.


Accordingly, spectral TDE velocities are usually obtained from the apical acoustic window, since LV longitudinal velocities are less affected by translational motion ( Figs. 12-3 and 12-4 ). Alternatively, translational motion may be corrected by offline analysis from color-encoded TDE velocities. One method plots the velocity of each adjacent scan line from the distance of the epicardium to the endocardium. From a parasternal color M-mode image, the rates of circumferential fiber shortening and lengthening are proportional to the slope of the velocity/distance regression line. The value of this slope has been referred to as the myocardial velocity gradient (MVG).


MYOCARDIAL STRAIN AND STRAIN RATE
The change in length of the myocardial fiber (end diastolic length, end systolic length/end diastolic length) is also known as myocardial strain. The spiral architecture of the myocardial fiber bundles determines strain deformation in multiple directions. Thus, changes in LV geometry during LV systole relate primarily to radial (short axis), longitudinal (long axis), and meridional (LV torsion) strain ( Fig. 12-5 ). Myocardial strain may be quantified noninvasively using magnetic resonance imaging (MRI), TDE, or high-frame-rate two-dimensional echocardiography with speckle tracking. (Strain analysis using MRI is described in detail in a separate chapter.) TDE-derived strain quantifies tissue deformation based on Doppler velocity shifts. Unlike tissue Doppler velocities, tissue strain is less affected by segmental tethering and translational motion; therefore, it reflects primarily the intrinsic deformation of the myocardial segment within the sample region. The strain rate (SR) of a segment of a given length (L 0 ) is given by: SR = (V 1 − V 2 )/L 0 , where V 1 and V 2 are the tissue Doppler velocities at each end of the segment (L 0 ). Strain (ε) represents the percent deformation for the given segment over its initial value, and is obtained by integrating SR over the systolic (shortening) or diastolic interval (lengthening) of the cardiac cycle ( Figs. 12-6 and 12-7 ). Recent studies have demonstrated a closed correlation between SR and indices of LV contractility and between ε and LV stroke volume. Unfortunately, Doppler-derived strain is limited to interrogating segments aligned in parallel with the Doppler angle of incidence. More recently, strain analysis derived from two-dimensional speckle tracking has become available. This method promises to be more robust than Doppler-derived strain, and may provide strain analysis in multiple directions, from virtually any echocardiographic image.



LEFT VENTRICULAR TORSION
In addition to radial and longitudinal deformation, there is torsional deformation of the left ventricle during the cardiac cycle due to the helical orientation of the myocardial fibers. During systole, the basal segments of the LV myocardium rotate or twist in counterclockwise direction, whereas the apical segments twist in clockwise direction. During diastole, untwisting occurs in the opposite direction. Systolic torsion represents the net effect of basal and apical twist. Apical twisting is the main component of global LV systolic torsion, and in the next diastole, the apical untwisting also plays the dominant role, whereas basal rotation is of less importance. Torsional deformation may be quantified by MRI or by either TDE velocities or high-frame-rate two-dimensional echocardiography with speckle tracking ( Fig. 12-8 ). Systolic LV torsion tends to equalize sarcomere shortening between endocardial and epicardial layers of the left ventricle and is a possible mechanism by which potential energy can be stored during ejection and then released during early diastole to generate suction and rapid filling by “elastic recoil.”

LV untwisting has been shown to be dissociated from filling and accentuated by catecholamines, with the untwisting rate related to LV pressure decay. The magnitude of the restoring force appears to be inversely related to end systolic volume. Epicardial contraction dominates the direction of torsion because these fibers are at larger radii and therefore produce greater torque than do those in the inner layers. The process can be viewed as a global LV spring between the epicardium and endocardium that is stretched during systole, storing potential energy for release in early diastole. Accordingly, LV myocardial contraction and relaxation are tightly interconnected: The potential energy stored during systole is converted to kinetic energy during diastole, thus effectively bridging the two periods. Peak LV untwisting velocity occurs approximately 60 msec earlier than long-axis lengthening and short-axis expansion and may be accentuated by catecholamine infusion. Left ventrical untwisting is 40% to 50% completed by the time of mitral valve opening and continues during early LV filling.
CLINICAL APPLICATIONS
Doppler myocardial velocities and, to a lesser extent, strain analysis have been used to evaluate myocardial relaxation in a variety of cardiac diseases associated with LV diastolic dysfunction. In addition, TDE combined with LV filling Doppler indices has been used to evaluate LV filling pressures.
Assessment of Left Ventricular Relaxation
Several studies have shown an inverse relationship between E M and LV relaxation (τ) in patients with both normal and elevated preload. Tissue Doppler E M has been shown to be less influenced by preload alterations than standard Doppler LV filling indices, particularly in the presence of slow ventricular relaxation. Clinical studies suggest that E M is a better discriminator between diastolic dysfunction and normal patients, compared with any other single or combined index of transmitral filling and pulmonary venous Doppler flows. LV torsion and untwisting also correlate well with the relaxation time constant and with the early LV diastolic intraventricular pressure gradient (IVPG) (that is, LV suction). Delayed LV untwisting has been reported in patients with severe aortic stenosis and impaired LV relaxation. Enhanced LV torsion during exercise is associated with increased IVPG. We have recently demonstrated that the ability to increase IVPG during exercise is directly related to aerobic capacity in normals and in heart failure patients.
Evaluation of Left Ventricular Filling Pressures
Several investigators have studied the utility of Doppler ECG for the assessment of LV filling pressures. Previous studies have demonstrated a relationship between mean LA pressure and pulmonary venous systolic (S)/diastolic (D) ratio, mitral E/A ratio, isovolumic relaxation time (IVRT), and deceleration time (DT). All pulsed Doppler–based methods are accurate when applied to groups of patients with homogeneously impaired LV relaxation since they assume that reduction in IVRT, atrial filling fraction, pulmonary venous S/D ratio, and DT will occur solely as a consequence of elevated LA pressure. However, when these methods are applied to younger patients and those with minimal structural heart disease with a normal ejection fraction (EF), they overestimate actual LV filling pressure since they cannot separate the effect of LV relaxation and preload as confounding variables. TDE velocities used as an index of LV relaxation may be combined with standard Doppler flow indices in order to separate these confounding effects. Since pulsed Doppler E velocity is determined equally by both LA pressure and LV relaxation, whereas TDE E M is related primarily to LV relaxation, the ratio of E/E M may be used to predict LA pressure. This concept has been developed and validated in relatively heterogeneous groups of patients undergoing right heart catheterization. In subjects with normal LV relaxation and normal LA pressure, both E and E M are elevated. In subjects with impaired relaxation and normal LA pressure, both E and E M are decreased. In patients with impaired relaxation and elevated LA pressure, E is elevated but E M is reduced. An E/E M ratio greater than 15 is almost invariably associated with a mean LA pressure greater than 15 mmHg.
The combined E/E M ratio also appears to be an important independent predictor of exercise capacity. In 121 subjects who were studied with Doppler echocardiography before maximal exercise testing, exercise capacity was similar in the population with a normal mitral inflow pattern (E/A >1) and those with a slow relaxation pattern (E/A <1) only when E/Ea was less than 10 ( Fig. 12-9 ). However, those subjects with slow relaxation and E/Ea less than 10 had reduced exercise tolerance. Compared with other echocardiographic and clinical parameters, E/Ea had the best correlation with exercise capacity ( r = 0.684, p < 0.001) and was the strongest independent predictor of exercise capacity less than 7 metabolic equivalents (METs) by multivariate analysis.

Ischemic Heart Disease
Ischemia affects relaxation by limiting the availability of energy substrates in the form of adenosine triphosphate (ATP). The reabsorption of Ca ++ ions by the sarcoplasmic reticulum is an energy-dependent process, which is required for the deactivation of the troponin-tropomyosin complex. In the presence of normal systolic function at baseline, ischemia is manifested by a change to a pattern of impaired LV relaxation. Doppler LV filling patterns will vary according to the extent, duration, and severity of ischemia. These patterns have been shown to carry important prognostic information after an acute myocardial infarction and in chronic ischemic heart disease. TDE myocardial velocities and strain indices may identify myocardial ischemia and viability during pharmacologic stress testing ( Fig. 12-10 ). Strain rate imaging may be used to identify not only the magnitude but also the time of peak systolic myocardial contraction. Postsystolic shortening is a sensitive marker of ischemia. In pigs subjected to acute myocardial ischemia during angioplasty balloon inflation, there is a strong direct correlation between the spatial distributions of postsystolic shortening measured by strain rate imaging and myocardium at risk. In patients with ischemic cardiomyopathy, an increase of peak systolic strain rate from rest to dobutamine stimulation by more than −0.23 s- allowed accurate discrimination of viable from nonviable myocardial segments, as determined by 18-fluorodeoxyglucose positron emission tomography with a sensitivity of 83% and a specificity of 84%. In this study, strain imaging was superior to TDE and two-dimensional wall thickening subjective assessment.
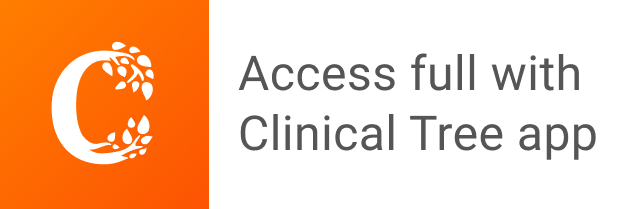