Fig. 15.1
Epigenetic regulators modify histone tails of a nucleosome. Epigenetic factors can be categorized into “writers,” “erasers,” and “readers.” Writers add specific modifications to the histone tails of a nucleosome, while erasers remove these modifications. Readers function to recognize epigenetic modifications and can recruit other regulatory factors. Examples of each class of epigenetic regulator are provided
15.2 Epigenetic Changes During Cardiac Development
The explosion of next-generation sequencing technologies coupled with the improved efficiency of directed differentiation of embryonic stem cells into cardiac lineages has enabled mapping of the landscape of histone modifications over the course of cardiac differentiation. Most prominently, work by Paige et al. and Wamstad et al. have shed significant light on the dynamics of gene activation and repression during cardiac specification [2, 3]. By taking snapshots at a series of well-defined time points in mouse and human ESC differentiation, these groups defined the presence of various histone modifications across the genome during cardiogenesis using chromatin immunoprecipitation coupled with massively paralleled sequencing (ChIP-Seq). Specifically, they observed unique histone modifications marking transcription start sites (trimethylation of histone H3 at lysine 4; H3K4me3), enhancers (monomethylation of histone H3 at lysine 4; H3K4me1, and acetylation of histone H3 at lysine 27; H3K27ac), and inactive chromatin (trimethylation of histone H3 at lysine 27; H3K27me3), thus providing an initial “map” of the dynamic epigenetic landscape during cardiac development. A theme that emerges from these surveys is that genes specific to noncardiac lineages such as endoderm and ectoderm derivatives are rapidly marked by repressive histone modifications, resulting in repression of these lineages. These findings support the hypothesis that cell differentiation requires active silencing of alternative lineages.
These studies also suggest that cardiac-specific enhancers are marked by H3K4me1 [3]. Integration of these epigenetic maps with publicly available databases will guide further studies of epigenetic changes during cardiac development.
15.3 Histone Modifications
Epigenetic studies have focused on understanding how histone proteins undergo posttranslational modifications, which amino acid residues are subject to these modifications, and how various combinations of modifications affect gene expression. Methylation and acetylation are two of the most common and well-studied histone modifications. Histones can be methylated on a variety of residues and Jumonji domain-containing proteins act as demethylases. Histone acetyltransferases (HATs) acetylate lysine residues primarily on histone tails, and histone deacetylase enzymes (Hdacs) reverse this process. Additional forms of histone modification have been described and continue to be discovered, though application to cardiac development and disease awaits further study [4].
15.3.1 Histone Deacetylase Enzymes
Histone deacetylases (Hdacs) remove acetyl groups from lysine residues, typically resulting in compaction of chromatin and repression of gene expression. Histones represent only one type of the many possible substrates for Hdacs, which can also remove acetyl groups from lysine residues of many other proteins, including tumor protein 53 (p53) and GATA binding protein 4 (Gata4) [5, 6]. Hence, these enzymes are more accurately known as lysine deacetylases (Ldacs) and nonhistone targets likely account for many important developmental functions that remain to be fully elucidated. There are five classes of Hdac proteins: I, IIa, IIb, III, and IV. Class I includes Hdacs 1, 2, 3, and 8, and these enzymes are expressed in most tissues [7–9]. Hdacs 1 and 2 are members of several repressive complexes including the Sin3 deacetylase, repressor element-1 silencing transcription factor corepressor 1/deacetylase (CoREST), nucleosome-remodeling deacetylase (NuRD), and polycomb-repressive complex 2 (PRC2) complexes [10]. Hdacs 4, 5, 7, and 9 comprise the class IIa subfamily of Hdacs. Class IIa Hdacs exhibit minimal deacetylase activity in vivo and in vitro due to evolutionary divergence of a critical tyrosine residue within the catalytic pocket. These Hdacs are characterized by a highly conserved myocyte enhancer factor 2-binding domain (Mef2). In addition, class IIa Hdacs act as signal integrators through interactions with 14-3-3 chaperone proteins to facilitate shuttling in and out of the nucleus [9]. Class III sirtuins require NAD+ for deacetylation activity and have been studied in the context of cardiac hypertrophy [11, 12]. Hdac11 is the sole member of class IV and is expressed in the heart; however, its function remains poorly described [13].
15.3.1.1 Hdac1 and Hdac2
Hdac1 and Hdac2 share a large degree of amino acid homology, are thought to have mostly redundant functions, and are both expressed in the myocardium [14, 15]. Global Hdac1-null mice die in utero at approximately E10.5, but myocardial specific deletion of Hdac1 does not result in gross cardiac abnormality [14]. Global Hdac2 deletion by a gene trap allele results in partial lethality, but surviving mutants exhibit only slightly thickened myocardial walls under homeostatic conditions [15]. However, these mutant mice are resistant to the cardiac hypertrophy that results fom β-adrenergic stimulation or transaortic constriction. Montgomery et al. generated a floxed allele of Hdac2, and global deletion of Hdac2 (by crossing the floxed allele to a cytomegalovirus-Cre recombinase mouse) results in lethality of all mice within 24 h of birth [14]. The differences between the phenotypes of the gene trap and floxed alleles are likely due to differences in genetic background and/or efficiencies of Hdac2 inactivation. Combined deletion of Hdac1 and Hdac2 in cardiomyocytes results in death within 2 weeks of birth due to cardiac arrhythmias and cardiomyopathy, along with ectopic expression of fetal ion channels and contractile proteins [14]. It should be noted that compound deletion of Hdac1 and Hdac2 just a few days earlier in cardiac development leads to intrauterine demise, highlighting the changing role of Hdacs during cardiac development, especially during critical times of progenitor cell commitment, proliferation, and differentiation.
15.3.1.2 Hdac3
Like other class I Hdacs, Hdac3 is expressed in the myocardium throughout development and into adulthood. Germline deletion leads to lethality during gastrulation, before the heart is formed, and any cardiac phenotype can be appreciated [16]. Specifically, genetic deletion of Hdac3 in early cardiac progenitors results in embryonic lethality late in gestation and ventricular hypoplasia [17]. Hdac3 acts as a repressor of T-box transcription factor 5 (Tbx5), a known driver of cardiogenesis, which also plays critical roles in development of the cardiac conduction system and atrial septation [5, 18, 19], though it is unclear if deacetylation of Tbx5 protein by Hdac3 is required for cardiogenesis. Myocyte specific deletion of Hdac3 during mid-late gestation with alpha–myosin heavy chain-Cre recombinase (αMHC-Cre) permits survival until 3–4 months of age when mice succumb to severe cardiac hypertrophy and fibrosis [20]. Interestingly, deleting Hdac3 in postnatal myocardium and skeletal muscle with myosin creatine kinase-Cre (MCK-Cre) results in a very mild phenotype and no lethality unless the mice are stressed by a high-fat diet [21]. Switching the mice from normal chow to a high-fat diet results in severe hypertrophic cardiomyopathy and fibrosis followed by death of all animals within several weeks. Myocyte specific deletion of Hdac3 is also characterized by extensive metabolic dysregulation of both lipid and glucose processing. Consistent with these findings, Hdac3 is unique among Hdacs for its interaction with the nuclear receptor corepressor-silencing mediator for retinoid and thyroid receptor (NCoR/SMRT) complex that has been previously implicated in metabolic regulation [22–24].
Hdac3 is also a potent regulator of cell proliferation and directly regulates p21 [25, 26]. Previous work in transformed cells and hematopoiesis implicated Hdac3 in regulating cell cycle progression and DNA replication [26, 27]. Transgenic overexpression of Hdac3 in cardiomyocytes causes increased proliferation and ventricular hyperplasia [25]. Expression analysis indicated that Hdac3 represses several cyclin-dependent kinase inhibitors, reducing levels of these cell cycle checkpoints and promoting rapid proliferation of cardiomyocytes.
15.3.1.3 Hdac5 and Hdac9
These two class II Hdacs are expressed in the myocardium and skeletal muscle, and individual genetic loss yields viable adult mice without an overt cardiac phenotype under homeostatic conditions [28, 29]. However, combined deletion of Hdac5 and Hdac9 results in sensitivity to hypertrophic signals, phenotypically opposite to that of Hdac2-null animals. Loss of Hdac5/9 leads to overactive Mef2 signaling in response to calcineurin-dependent signaling, which normally leads to export of class IIa Hdacs from the nucleus and inhibition of Mef2 activity. The absence of Hdac5/9 leaves Mef2 within the nucleus where it can activate hypertrophic gene programs [29]. Class IIa Hdacs also modulate other transcription factor complexes, including serum response factor (SRF), myocardin, and calmodulin-binding transcription activator 2 [30], and it is likely that deletion of both Hdac5 and Hdac9 has effects on these regulators and Mef2 throughout development.
15.3.1.4 Hdac7
Global deletion of Hdac7 leads to midgestation death at E11.5 due to vascular defects [31]. Mutant mice have vascular leak and lack smooth muscle cells around the dorsal aorta. Hdac7 complexes with Mef2 to repress matrix metalloproteinase 10 (Mmp10) in endothelium, which has been shown to influence the integrity of surrounding smooth muscle [31]. Consistent with a primary role of Hdac7 in endothelial cells, knockdown studies of Hdac7 in endothelial cell cultures results in abnormal cellular morphology and tube formation [32].
15.3.1.5 Sirtuins
Sirtuins are class III Hdacs and require nicotinamide adenine dinucleotide (NAD) for their catalytic activity. There are seven sirtuins in mammals with various expression patterns, cellular localizations, and functions [11, 12]. Sirt1 is the best-studied sirtuin and is located in the nucleus and cytoplasm [33, 34], while Sirt2 is restricted to the cytoplasm [35]. Sirt3, Sirt4, and Sirt5 are localized to the mitochondria primarily [36]. Sirt6 and Sirt7 are localized to the nucleus [37, 38]. Sirt6 is involved in deacetylation of H3K9 and H3K56 [38], while Sirt7 modulates gene transcription dependent on RNA polymerase-I [37]. Multiple sirtuin proteins have been implicated in the pathogenesis of cardiac hypertrophy, but to our knowledge, there is no clear role for sirtuins in the most common forms of congenital heart disease.
15.3.2 HAT Proteins: Histone Acetyltransferases
Histone acetyltransferase enzymes catalyze the addition of acetyl groups to lysine residues of histone tails. This results in relaxation of chromatin, greater accessibility of the transcriptional machinery to DNA, and an increase in gene expression.
The most well-studied HAT is p300, with genetic deletion resulting in a generalized lack of cell proliferation, myocardial thinning, and death between E9.5 and E11.5 [39]. A single amino acid mutation abrogating the acetyltransferase activity results in myocardial thinning as well, but these mutants live to E12.5–E15.5 [40]. p300 also acetylates nonhistone proteins, including Gata4, which plays multiple roles in cardiac myocyte specification, differentiation, and proliferation [5, 41]. Acetylation of Gata4 enhances its transcriptional activity [42]. Interestingly, Hdac2, functioning with the atypical homeodomain protein, Hopx, can deacetylate Gata4 and represses its transcriptional activity [5]. This pathway is important in cell cycle exit and reducing the proliferative capacity of myocytes in late gestation. These are examples of traditional “epigenetic” factors acting on nonhistone proteins to indirectly affect cardiac gene expression.
15.4 Histone Methylation
Multiple arginine and lysine residues on histone proteins are methylated. Gene activation or gene repression is dependent on which residue of the histone tail is methylated. Gene activation is associated with methylation at lysine (K) 4, K36, and K79 of histone H3, while gene repression is associated with methylation of K9 and K27 [4]. To date, histone methyltransferases and demethylases have not been studied as comprehensively in the context of cardiac development as Hdac and HAT proteins.
15.4.1 Jumonji Proteins
Methyl groups are removed from histone tails by a family of proteins containing JumonjiC-domains. Two members of this family, jumonji AT-rich interactive domain 2 (Jarid2) and jumonji domain-containing 6 (Jmjd6), have important roles in cardiac outflow tract septation. Jarid2 is expressed in the developing myocardium of mice, as early as E8.0. The developing outflow tract, ventricular septum, and ventricular walls express relatively high levels of Jarid2 as compared to the atria. Jarid2 remains expressed in the myocardium in the adult mouse but at lower levels than during development. Global deletion of Jarid2 results in hypertrabeculation of myocardium and outflow tract defects, including double outlet right ventricle (DORV) [43, 44]. Jarid2 controls expression of Notch1, and its downstream target, Neureglin1, both implicated in the control of trabecular growth [44]. Similarly, Jmjd6 knockout mice demonstrate DORV [45]. The nature of the defects suggests that these proteins affect neural crest function, though conditional deletion in the neural crest will be necessary to establish the tissue-specific roles of these proteins.
15.4.2 WHSCI1 and Wolf-Hirschhorn Syndrome
Wolf-Hirschhorn syndrome is characterized by mental retardation, epilepsy, craniofacial abnormalities, and myocardial septal defects. Genetically, the syndrome is correlated with mutations and/or translocations of WHSC1 (Wolf-Hirschhorn syndrome candidate 1), which encodes a histone methyltransferase that specifically methylates H3K36 and H4K20 [46]. Knockout Whsc1 mice are characterized by myocardial septal defects, bone defects, and growth delay. However, haploinsufficiency of Whsc1 in murine models does not result in the overt features associated with the clinical syndrome [47]. However, Whsc1 is known to associate with NK2 homeobox 5 (Nkx2-5), and compound haploinsufficiency of Whsc1 and Nkx2-5 in murine models recapitulates the atrial and ventricular septal defects associated with the clinical syndrome [47]. Some human mutations involve deletions of large pieces of chromosome 4, including the nearby Msh Homeobox 1 (MSX1) gene, which is highly expressed in the mesenchyme that contributes to craniofacial structures including teeth. Some of the typical craniofacial features of Wolf-Hirschhorn syndrome, such as dental abnormalities and cleft lip and/or palate, are more prominent in patients when MSX1 is lost as well [48].
15.4.3 Smyd Proteins
The SET and MYND (Smyd) proteins are a family of five proteins characterized by a SET domain, which catalyzes methyltransferase activity, and a protein–protein interaction MYND domain [49]. The MYND domain allows Smyd proteins to interact with Hdacs, and Smyd1 represses gene transcription in an Hdac-dependent manner [50]. Interestingly, multiple Smyd family members have been detected in the cytoplasm and can be shuttled into the nucleus. This suggests that Smyd proteins likely have nonnuclear functions such as methylation of nonhistone proteins [49]. Smyd1 is the founding member of this family of proteins, and knockout studies in mice demonstrate a critical role in cardiac myocyte maturation and morphogenesis of the right ventricle [50]. This defect may be due to, at least in part, Smyd1 regulation of Heart and Neural Crest Derivatives Expressed 2 (Hand2) and Iroquois Homeobox 4 (Irx4) expression [50]. Zebrafish studies confirm a critical role for smyd1b, one of two zebrafish orthologues; knockdown of smyd1b results in mispatterning of myofibers and lack of myocardial contraction [51, 52]. Smyd2, known to associate with the Sin3 complex [53], is strongly expressed in the embryonic myocardium and highly related to Smyd1 [54]. Smyd2 knockdown in zebrafish results in cardiac dysfunction and myocyte disorganization [55]. However, knockout studies in mice demonstrate Smyd2 is dispensable for normal cardiogenesis [54]. Smyd3 knockdown in zebrafish results in abnormal cardiac looping and defective expression of essential myocyte genes [56].
15.4.4 MLL2 and Kabuki Syndrome
Kabuki syndrome is an autosomal dominant syndrome characterized by mental retardation, distinctive craniofacial abnormalities, skeletal anomalies, and short stature [57]. The incidence of congenital heart disease in patients with Kabuki syndrome is 30–55 %. There is a wide spectrum of reported defects including aortic coarctation, septal defects (atrial and ventricular), and hypoplastic left ventricle [58, 59]. Type I Kabuki syndrome is associated with mutations in the mixed-lineage leukemia protein 2 (MLL2), a histone methyltransferase belonging to the Trithorax family of proteins [60]. MLL2 is required for methylation of H3K4 [61, 62]. Mutations in MLL2 have been detected in 48–75 % of Kabuki patients, and nonsense or frameshift mutations are the most common types of mutations detected. Type II Kabuki syndrome, a more rare form of the disorder, is associated with mutations in KDM6A, a histone demethylase that specifically acts on H3K27 [63].
15.5 Epigenetic Gene Variants and Sporadic Congenital Heart Disease
The study by Zaidi et al., as part of the Pediatric Cardiac Genomics Consortium, provided a fundamental advance in our understanding of epigenetics and congenital heart disease by sequencing the exomes of 362 trios (affected patients and their parents) with a variety of congenital heart disorders, along with a cohort of 264 unaffected trios [1]. Interestingly, there was an overrepresentation of mutations or variants in genes encoding epigenetic enzymes in affected patients when compared to unaffected controls. Cohorts were largely composed of patients of European ancestry. Probands were affected by a variety of forms of congenital heart disease, but mostly composed of conotruncal defects (n = 153 patients, transposition of the great arteries, DORV, TOF, partial truncus arteriosus, and aortic arch abnormalities), LV obstructive lesions (n = 132, hypoplastic left heart syndrome, aortic coarctation, bicuspid aortic valve), and heterotaxy (n = 70). Extracardiac abnormalities including craniofacial defects were present in 22 % of probands. The authors identified mutations predicted to be pathologic in 28 genes. These predictions depended on whether the mutations resulted in premature termination, splicing variants and/or frameshift of the coding sequence. The analyses also demonstrated that these mutated genes were amongst the highest expressed genes in the developing heart (25 % percentile or higher). Mutations in five genes were specifically associated with histone methylation of H3K4: MLL2, WDR5, CHD7, KDM5A, and KDM5B. H3K4me is known to mark active promoters and genes “poised” to be expressed. MLL2, as previously discussed, deposits the methylation of H3K4 [62]. JARID1A and JARID1B are responsible for removing methyl groups on H3K4, and CHD7 recognizes this histone modification [64]. Additionally, WD repeat domain 5 (WDR5) has been identified as part of the MLL complex [65]. Interestingly, the proband identified with a CHD7 mutation did not manifest typical features of CHARGE syndrome, though a patient with MLL2 mutation did display characteristic craniofacial abnormalities typical of Kabuki syndrome. The group also identified mutations in ring finger protein 20 (RNF20) and ubiquitin-conjugating enzyme E2B (UBE2B), factors involved in the ubiquitination of H2BK120, which is required for H3K4 methylation.
15.6 DNA Methylation
In addition to histone proteins, the C5 position of cytosine nucleotides can be directly methylated (5-mC). The DNMT family of proteins (DNA methyltransferases) is composed of three proteins that catalyze transfer of methyl groups onto DNA in CpG islands. DNMT1 recognizes previously methylated DNA to reinforce existing patterns after DNA replication. DNMT3a and DNMT3b are de novo DNMTs and catalyze methylation of previously unmethylated cytosines [66]. More recently, it was discovered that the methylcytosine dioxygenase (TET) family of proteins catalyzes DNA demethylation through the conversion of 5-mC to 5-hmC as well as 5-formylcytosine (5fC) and 5-carboxylcytosine (5caC) [66]. Methyl-CpG-binding domain proteins, including MeCP2, recognize methylated DNA and recruit histone-modifying complexes. Mutations in MECP2 have been implicated in the pathogenesis of Rett syndrome, a condition characterized by regression of skills, significant neurological difficulties, and stereotypical movements [67]. However, it was recently determined that approximately 20 % of patients with Rett syndrome have a prolonged QT interval on their electrocardiogram, which can lead to sudden cardiac death in some patients [68]. Further work is needed to determine if mutations in TET proteins or DNMTs in humans are associated with congenital heart disease.
15.7 Chromatin Architecture
The organization and three-dimensional structure of chromatin has emerged as a significant regulator of gene transcription. Dense, heterochromatic regions are associated with repressed gene activity and euchromatic regions with a more open conformation permit increased access by transcription factors to the DNA and are associated with increased gene transcription. Regulating higher order chromatin structure in this way allows for simultaneous control of large genomic regions and coordination of gene expression – a requirement for large-scale processes such as cell differentiation and stress response. For example, epigenetic mapping has demonstrated that as cells exit pluripotency during development and become more lineage restricted, levels of heterochromatin increase and the genome becomes more compact while cell fates stabilize [69, 70]. Chromatin-remodeling complexes such as Brg1-associated factor (BAF), CHD, and inositol requiring 80 (INO80) contain ATPase domains that catalyze nucleosome restructuring–detaching histone proteins from the DNA helix and reassembly of the complex elsewhere. In addition, these complexes may contain domains for sensing histone modifications or other epigenetic marks and reorganize the chromatin accordingly. Finally, emerging evidence has also identified three-dimensional positioning of chromatin within the nucleus as an additional layer of regulation of chromatin structure and transcriptional activity.
15.7.1 ATP-Dependent Chromatin Remodelers
Chromatin remodeling complexes are a class of “writers” that are characterized by SWI-like ATP-dependent catalytic activity. There are several classes of chromatin remodeling complexes including the switching defective/sucrose nonfermenting (SWI/SNF), chromodomain, helicase, DNA binding (CHD), and INO80 complexes. These complexes all contain a core ATPase domain that serves to disassemble the DNA-histone contacts to restructure and reposition nucleosomes. The complexes also serve as bridges to other regulatory factors and readers of histone modifications, making them important regulators of chromatin architecture and organization.
15.7.2 SWI/SNF Complex
The SWI/SNF complex was identified and purified in yeast with a vertebrate homolog BAF composed of 12 subunits. The core ATPase subunit is encoded by one of two highly homologous genes, SWI/SNF-related, matrix-associated, actin-dependent regulator of chromatin, subfamily a, member 4 (also known as brahma-related gene 1; Brg1) and SWI/SNF-related, matrix-associated, actin-dependent regulator of chromatin, subfamily a, member 2 (Brm); however, most studies suggest that Brg1 is the dominant ATPase in cardiovascular development [71].
Brg1 is expressed from the onset of embryogenesis, and Brg1-null embryos do not advance past implantation [72]. Endothelial-specific deletion of Brg1 results in embryonic lethality at E10.5 and deficient yolk sac vasculogenesis [73]. Loss of Brg1 in the endocardium results in hypotrabeculation. Further analysis revealed that Brg1 acts to repress a metalloproteinase, Adamts1, responsible for breakdown of the cardiac jelly present in the early embryonic heart. Loss of Brg1 allows premature expression of Adamts1, early degradation of the cardiac jelly, and lack of normal trabeculation. Deletion of Brg1 in cardiac myocytes results in reduced cell proliferation and thin compact myocardium, ventricular septal defects, and lethality at E11.5 [71]. In developing cardiomyocytes, Brg1 complexes with Hdacs and poly (ADP-ribose) polymerases (PARP) to repress the adult isoform of myosin heavy chain and activate the fetal form, permitting normal embryogenesis. Loss of Brg1 induces premature expression of the adult myosin isoform and leads myocytes to exit the fetal state too rapidly. Conditional deletion of Brg1 results in hypoplastic right ventricle, shortened outflow tract, and embryonic lethality at E11.5 [71]. Smooth muscle depletion of Brg1 leads to reduction of contractile proteins and dilated cardiomyopathy secondary to patent ductus arteriosus [74–77]. Taken together, these tissue-specific deletions suggest that Brg1 is an essential regulator of cardiac development as a core ATPase of the BAF complex, and its abrogation can lead to a variety of congenital heart defects in animal models.
Polybromo 1 (Baf180) is another subunit of the BAF complex critical for normal cardiac development. Baf180 contains six bromodomains and serves as a “reader” of acetylated histone tails. Global deletion of Baf180 is embryonic lethal by E15.5 with ventricular hypoplasia and ventricular septal defects [78]. Epicardium-specific deletion disrupts normal vasculogenesis, resulting in coronary artery abnormalities [79]. Further analysis reveals that Baf180 is required for normal expression of elements of the retinoic acid pathway, a core regulatory mechanism of myocardial patterning. D4, zinc and double PHD fingers, family 3 (Baf45c or Dpf3) is a BAF subunit that also serves as a “reader,” in this case of histone tails modified by the addition of acetyl and methyl groups. Knockdown in zebrafish results in abnormal cardiac looping and poor cardiac function [80]. Notably, human patients with tetralogy of Fallot (TOF) exhibit increased expression of Baf45c in the right ventricle [81]. Cui et al. showed that Baf45c is also upregulated in the hypertrophied left ventricle of patients with aortic stenosis and hypertrophic cardiomyopathy [82]. Mechanistically, phosphorylation of Baf45c (Dpf3a) by casein kinase 2 induces hypertrophy by releasing HEY repressors from chromatin [82].
SWI/SNF-related, matrix-associated, actin-dependent regulator of chromatin, subfamily d, member 3 (Baf60c) has been shown to interact with Nkx2-5, Tbx5, and Gata4 to activate multiple genes critical for cardiogenesis [83]. Loss of function studies in mice and zebrafish have confirmed Baf60c regulates multiple steps of cardiogenesis including cardiac looping and morphogenesis [84–86]. Recent lineage tracing studies suggest that Baf60c-expressing cells give rise to the majority of the heart, in addition to extracardiac structures [87].
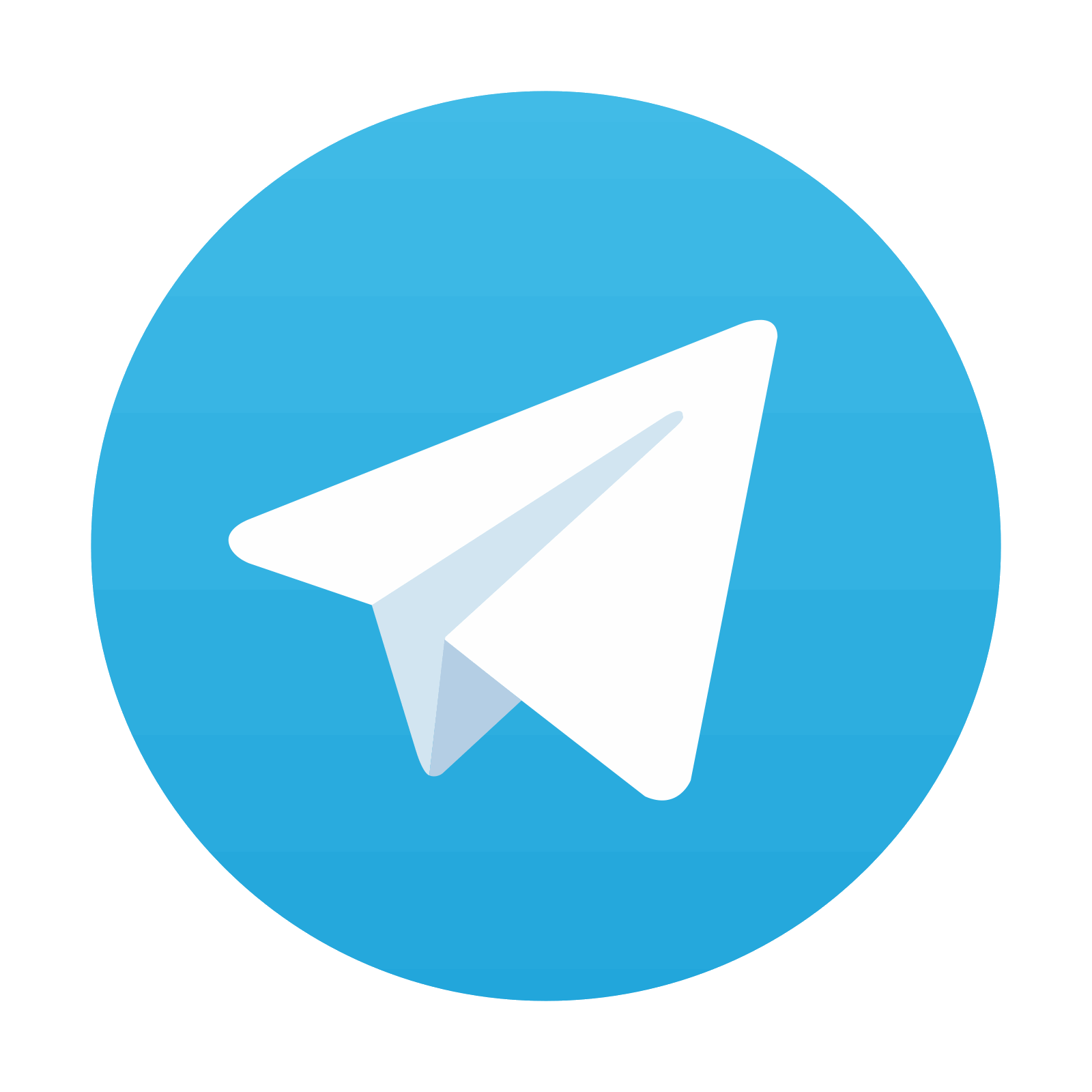
Stay updated, free articles. Join our Telegram channel
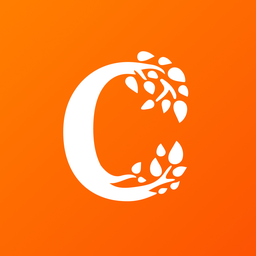
Full access? Get Clinical Tree
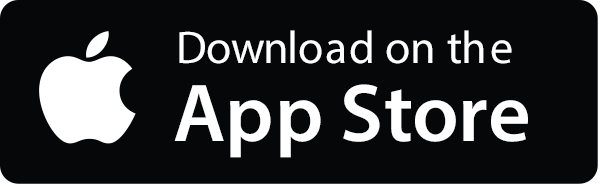
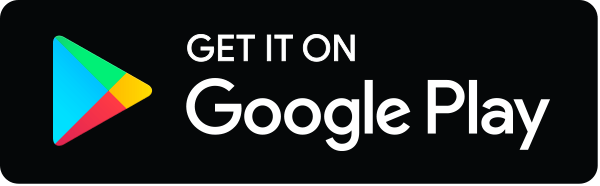