CHAPTER 5 Photoplethysmography (PPG) is a non-invasive optical technique that can be used to measure the real-time variation of clinically relevant physiologic parameters and extract physiological information from living organisms. In this chapter, we present experimental evidence demonstrating the effectiveness of PPG using multiple wavelengths, provide examples of clinically relevant applications, particularly the detection of oxygen content and blood flow in bone, and discuss the basic physics and engineering aspects of light-matter interactions that make PPG possible. PPG takes advantage of complex light-matter interactions occurring when light at visible or near-infrared wavelengths impinges upon, and interacts with, human tissues or organs.3, 19 The basic principle of PPG is that real-time changes of certain physiological parameters can affect physical processes governing the interaction of light with complex media (such as blood, skin, bone), causing a measurable, modulated variation in the fraction of light that is either reflected and/or transmitted. PPG has been used to study a number of biological tissues and organs in preclinical animal and human models including cardiovascular, lung, skin, muscle, and bone.2, 9, 22, 26 Its primary use has been to assess blood flow-related phenomena.9, 22 The features of PPG that contribute to its clinical utility are that it is non-invasive and that the AC component of the pulse wave is synchronous with the cardiac cycle. Essentially, PPG can detect blood volume changes in the circulatory system of several organs including capillary beds and, therefore, its clinical utility to date has been largely in circulatory diagnostics with a prime example being pulse oximetry.2 The simplest interpretation of the PPG waveform defines its applicability for circulatory measurements. The rising edge of the pulse (anacrotic) reflects systole and the declining edge (catacrotic) reflects diastole and wave reflections.2 Red, or near infrared wavelengths, are often chosen for the PPG light source since they are most compatible with the absorption spectra of blood and facilitate measurements of blood flow and blood volume.2, 16 Physiological measurements of circulation and oxygenation non-invasively in bone have been technically difficult. Currently, techniques that measure perfusion in soft tissues are not clinically applicable to bone since they cannot penetrate it (Doppler ultrasound), are invasive (laser Doppler and contrast MRI), or are destructive (fluorescent microspheres). Invasive techniques are unsuitable to screen for the presence of disease and cannot be used repeatedly to monitor responses to treatment. Laser Doppler flowmetry has a shallow penetration depth, typically around 1 mm, which limits its applicability to bone blood flow measurements without invasive probe placement or surgery. The ideal technique would be completely non-invasive and inexpensive enough to be used in a variety of clinical settings. Other techniques include photoacoustic imaging (PAI) and electrical impedance tomography (EIT). PAI uses non-ionizing lasers to generate a photoacoustic response and can reflect biological chromophores by optical absorbance.12, 31 EIT uses low frequency alternating currents to derive physiological information from the measurement of tissue conductivity. Both PAI and EIT have limitations in specificity and spatial field of view. PPG typically relies on few electronic components, e.g., a light emitting diode (LED) and a photodetector (PD), to record physiological changes related to blood circulation through light-matter interaction. PPG has been used to measure blood flow in human skin, muscle, and bone, but has not been applied extensively to monitor human bone diseases. The use of PPG in the context of bone blood flow measurement is heavily dependent upon the choice of appropriate wavelengths for the discrimination of intraosseous and intracutaneous blood flow. An adequate choice has to take into account the different optical properties of bone and skin at several wavelengths as well as their effects on light propagation in the tissues. Preliminary studies of the optical properties of bone have shown that it is nearly transparent to light in the near-infrared spectral range (800–1100 nm), thus providing a “biological window” for measuring blood flow. PPG takes advantage of this unique biological window to transcutaneously and non-invasively make several measurements of the interior of bone. Blood flow measurements by PPG also depend upon light absorption by blood and this absorption is different for oxygenated and deoxygenated hemoglobin. At a wavelength of ~808 nm, known as an isosbestic point, the absorption coefficients for oxygenated and deoxygenated hemoglobin are the same, enabling determination of total hemoglobin concentration irrespective of oxygen saturation levels13 (Fig. 5.1). Using different wavelengths, below and above the isosbestic point, the oxygenated and deoxygenated hemoglobin concentrations can be determined independently, permitting measurements of venous and arterial blood flow. A notable application is the determination of oxygen content and circulation in human brain made possible by the fact that bone is translucent in the 800–1,100 nm range.10, 15 Light with λ < 600 nm only penetrates about 1–2 µm and can be used to measure skin blood flow and develop a subtraction algorithm to improve sensitivity to bone perfusion. To test the feasibility of PPG for real-time measurement of blood flow, preliminary studies have been performed using a simple PPG optrode consisting of two LEDs with different wavelengths (532 nm and 850 nm, respectively) and a PD placed at a fixed separation distance from both LEDs. Figure 5.2 shows the use of two LEDs with wavelengths selected to preferentially sample the skin (green light) and arterial (infrared light) blood perfusion. The red light beam is able to penetrate deeper than the green beam into the tissue, thus enabling characterization of circulation beneath several tissue layers. Figure 5.3 shows an example of a typical PPG oscilloscope waveform originating from detection and processing of diffuse reflectance from the two wavelengths at separate time intervals. Both the green and infrared signals can be used to infer the heart rate of the patient (in this case, 67.4 beats per minute). However, the two signals differ substantially in their rise and decay times, with the green signal showing a slower response time while the red one shows a much faster response time. This difference arises from the fact that the two LEDs are sampling different regions. The red LED can access the arterial flow directly (therefore leading to a faster modulation of the light intensity upon interaction with arterial blood flow), while the green LED, being characterized by a shallower penetration depth, can only sample the superficial tissue layers and give information on the skin blood flow. As can be seen from Fig. 5.3, the green signal lags behind the red one, suggesting that there is a physiological delay between the vascular and skin perfusion. Moreover, the green signal shows slower rise and decay times, most likely indicative of the slower transit of blood through the skin capillary bed. Fig. 5.2. Two-color photoplethysmography (PPG) for detection of radial pulse as well as skin (green arrow) versus artery (red arrow) blood flows. The optrode is in contact with the skin, recording the superficial diffuse reflectance at each wavelength. Fig. 5.3. Measurement of heartbeat rate and blood flow using two light beams originating from LEDs with different wavelengths in the visible (green line, λ = 532 nm) and in the near-infrared (NIR, red line, λ = 850 nm). The “green” light beam samples the first superficial layers of the skin, thus detecting capillary skin blood flow, while the “red” light beam penetrates deeper into the tissue and is therefore able to sample the artery blood flow. Figure 5.4 displays an experiment in which arterial blood flow is occluded at time t = 2 seconds. Blood flow is then monitored in realtime for both skin (green) and artery (red) perfusion. The signal originating from the arterial blood flow (red) ceases almost immediately while the signal originating from interaction of light with skin blood flow shows a much slower decay time, due to a slower, measurable flow rate of skin perfusion. Both signals have finite, measurable time constants. Fig. 5.4. Two-color PPG experiment showing detection of blood flow “occlusion maneuver” at t = 2 s, as well as estimate of different time-constants for blood flow suppression in skin (slower, green line) and radial artery (faster, red line). Another experiment has attempted to quantify the perfusion changes occurring with a Valsalva maneuver. The two-color PPG experiment reported in Fig. 5.5 shows the ability of the PPG to quantify the heart rate change (in this case a decrease by ~10%), as well as changes in the skin and arterial blood flow caused by a physiological response induced by the maneuver. The preliminary experiments and results reported in Figs. 5.2–5.5 demonstrate the potential of two-color PPG as a quantitative technique for measurements of heart rate, blood flow, and real-time changes of blood perfusion in a human subject. PPG as an optical technique is based on inferring information on a complex medium based on its interaction with the incident light beam generated, for example, by a light emitting diode.23 In order to get a better sense of the potential as well as the limits of the technique, it is important to get a sense of the fundamental nature of these interactions. To this end, we adopt the quantized picture of light as a collection of elementary particles, named photons, incident upon a complex medium such as human skin or tissue. Each photon will interact with the medium according to the following physical processes: (a) Reflection
ENGINEERING AND CLINICAL ASPECTS
OF PHOTOPLETHYSMOGRAPHY
5.1 Introduction
5.2 PPG Measurements of Bone Circulation and Oxygen Content
5.3 Physiology of Multispectral PPG
5.4 Optical and Engineering Aspects of PPG
5.4.1 Introduction to light-matter interaction
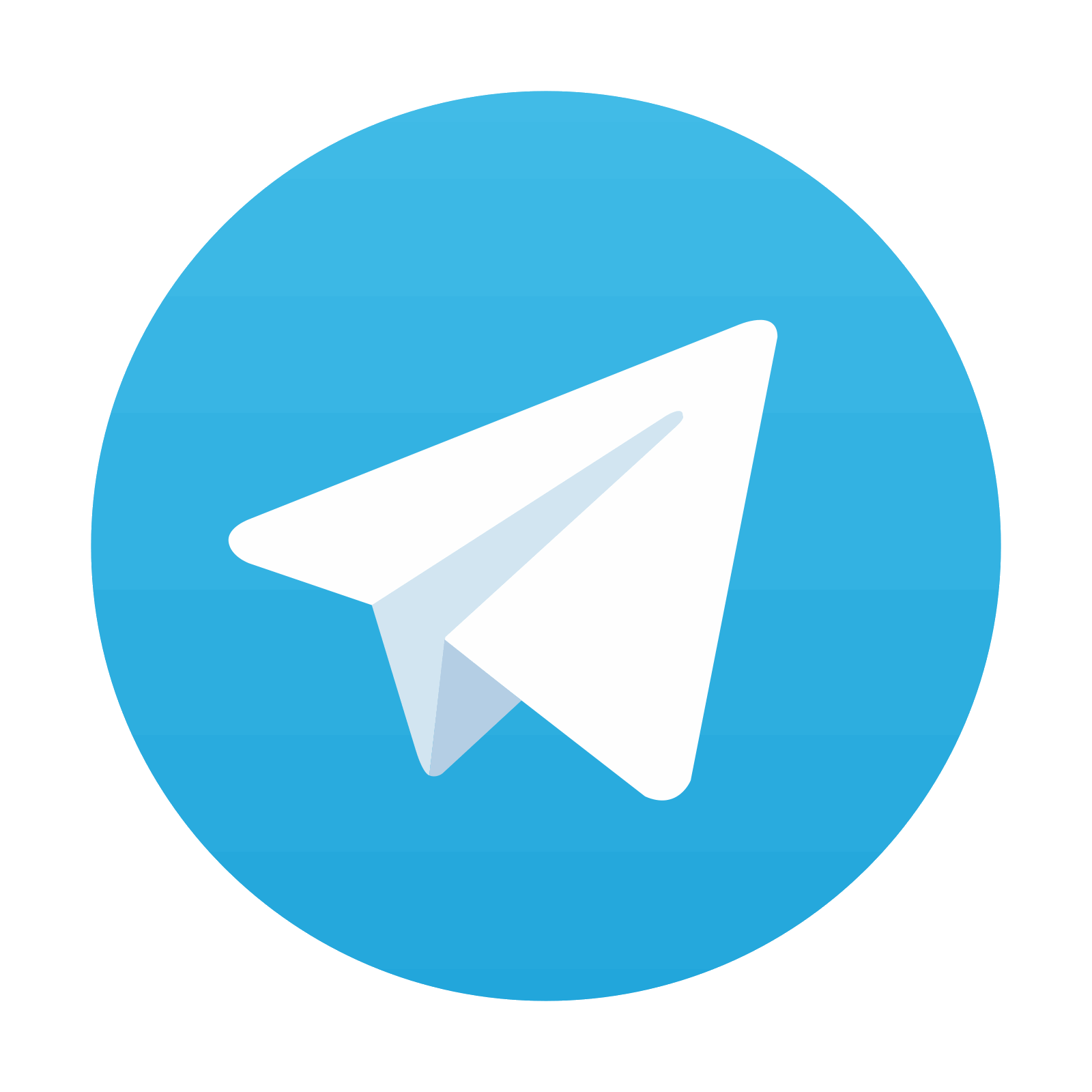
Stay updated, free articles. Join our Telegram channel
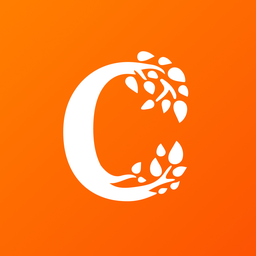
Full access? Get Clinical Tree
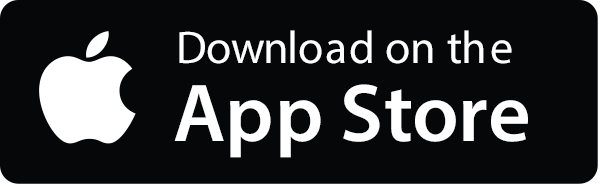
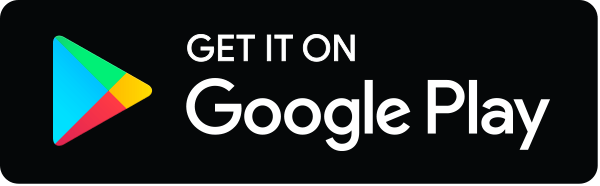