Endovascular Delivery of Gene and Stem Cell Therapy
Dara L. Kraitchman
Most preclinical studies of the efficacy of gene and stem cell therapies have relied on validation by postmortem tissue analysis using techniques, such as immunohistochemistry or polymerase chain reaction. These techniques can provide high spatial resolution information, which is microscopic, and, when used in combination with serial animal sacrifice, the distribution of stem cells or longevity of gene expression can also be obtained. However, noninvasive methodologies that can be used to serially assess the presence of stem cells or gene expression to determine the safety and the efficacy of these therapies would enhance clinical trials markedly. Thus, the introduction of magnetic resonance imaging (MRI) scanners with real-time imaging capabilities has expanded MRI from a diagnostic imaging tool to interventional applications directed at gene and cellular therapeutic delivery. However, x-ray angiography remains the imaging modality of choice because of the high temporal resolution for minimally invasive cardiac stem cell and gene delivery.
MR delivery of endovascular and transmyocardial therapies offers four major advantages over other imaging modalities: (a) The ability to acquire full three-dimensional (3D) images of the cardiovascular system with softtissue detail; (b) the lack of ionizing radiation for the interventionalist and the patient; (c) the lack of iodinated contrast agents that are often poorly tolerated by patients with cardiac or renal compromise; and (d) the ability to better target the therapeutic whether it be to determine viable myocardium using delayed contrast-enhanced MRI for stem cell targeting or vulnerable atherosclerotic plaques by MR vessel wall imaging for gene therapy targeting. In addition, the development of MR-visible labeling techniques offers a method to view targeted delivery and track the fate of these therapeutics over weeks to months.
Although computed tomography (CT) is emerging as a new technology for whole-body screening and rapid determination of stenotic coronary vessels and global left ventricular function, CT alone does not lend itself to endovascular delivery procedures. Nonetheless, the recent development of hybrid x-ray/MRI systems is an obvious extension that enables reductions in radiation dose while deriving the benefits of MRI along with the high temporal resolution and angiographic capabilities of x-ray imaging. As multislice CT scanners permeate the market, fusion of these 3D CT images with conventional x-ray angiography are becoming more routine for interventional procedures. The recent introduction of flat-panel x-ray detectors in place of traditional image intensifiers enables the acquisition of C-arm CTs, which are intrinsically registered with live x-ray fluoroscopic image (1), for 3D targeting and soft tissue detail. Fusion of live x-ray fluoroscopy with previously acquired CT, MRIs, and C-arm CTs is an enabling technology for stem cell and gene targeting on a platform that is familiar to the cardiac interventionalist using off-the-shelf devices.
ENDOVASCULAR MAGNETIC RESONANCE IMAGING DELIVERY DEVICES
In concert with the development of MR scanner with realtime acquisition capabilities, another obvious hurdle is the development of delivery devices that are MR-compatible. In the simplest form, a conventional x-ray delivery catheter
could be redesigned using nonmagnetic materials. However, often these MR-compatible devices either create large magnetic susceptibility artifacts or signal voids that either limit or prevent passive tracking of the device. At the other extreme, nonmetallic materials, such as nitinol guidewires or platinum stents, are essentially MR-invisible (Fig. 39.1), making passive tracking of these devices difficult (2).
could be redesigned using nonmagnetic materials. However, often these MR-compatible devices either create large magnetic susceptibility artifacts or signal voids that either limit or prevent passive tracking of the device. At the other extreme, nonmetallic materials, such as nitinol guidewires or platinum stents, are essentially MR-invisible (Fig. 39.1), making passive tracking of these devices difficult (2).
Several mechanisms for improving the passive visualization of these devices have been developed. A simple approach is to visualize a catheter lumen during injection of MR contrast agents similar to an x-ray angiogram (3,4 and 5). Another approach is to incorporate markers or coatings of paramagnetic or superparamagnetic iron oxide (SPIO) contrast agents in the devices (6,7,8,9 and 10) that can be tracked. Similarly, current-controlled susceptibility artifacts have also been used historically for tracking (11,12).
Active tracking approaches incorporate small MR receiver coil(s) into the device to receive the MR signal (13,14,15 and 16). In the simplest approach, a guidewire is used as a receiver coil or loopless antenna for imaging (17,18,19,20 and 21). The advantage of the active tracking approaches is the ability to obtain highresolution imaging from the miniaturized coils as well as the ability to perform catheter or catheter-tip localization by triangulation of several of these small coils (13,22,23). The cost of this improved resolution and localization is the increased complexity of both device manufacture and image acquisition control. In addition, increases in device diameter to accommodate the wiring from multiple coils and the increased risk of localized heating of the device have limited their use to preclinical animal studies. Several MR imaging guidewires have been safety tested and used in human clinical trials in transesophageal and transvenous approaches (Fig. 39.2) (24,25).
Two other approaches have emerged in the past 5 years to attempt to circumvent the concerns of heating of active MR interventional devices and poor handling characteristics of MR-compatible guidewires. One approach is based on transformers used in transmission lines for electric current where the receiver circuit is divided into short cables with miniature transformers to avoid long wire lengths that are prone to heating (26). The second approach is to create a polymer-based guidewire that is inherently nonferrous and has stiffness characteristics that mimic conventional guidewires. Krueger et al. (27) have developed a fiber-reinforced guidewire that is formed using micro-pultrusion methods. With handling characteristics that were very similar to
metallic guidewires, interventional MRI clinical trials were initiated (28). However, the lack of visualization of the micropultruded fiber-reinforced guidewire proved unsafe as the guidewire became severed in a patient during the early clinical trials. Another polymer guidewire made from polyetheretherketone that has an iron oxide coating for passive visualization is being tested preclinically for MRI-guided angioplasty and stenting (29).
metallic guidewires, interventional MRI clinical trials were initiated (28). However, the lack of visualization of the micropultruded fiber-reinforced guidewire proved unsafe as the guidewire became severed in a patient during the early clinical trials. Another polymer guidewire made from polyetheretherketone that has an iron oxide coating for passive visualization is being tested preclinically for MRI-guided angioplasty and stenting (29).
Specialized devices for stem cell injections under MRI guidance were developed by several device companies. A modified injection catheter system (Boston Scientific, Inc.), developed for x-ray-guided transmyocardial delivery of gene and stem cells, consisting of a curved guide catheter and spring-loaded injection needle, was modified for active catheter tracking and delivery in relevant large animal models of stem cell delivery for cardiac regeneration (30,31). A prototype steerable catheter with a nitinol needle for endovascular injections (Bioheart, Inc.) has been coated with gadolinium oxide markers for visualization under a hybrid x-ray/MR scanner (9). Using this hybrid imaging system, cardiac catheterization can be performed under x-ray guidance with accurate targeting to infarcted myocardium using MRI. A custom injection catheter has also been developed for stem cell therapeutic delivery with MR fluoroscopy (32) that enables access to all portions of the left ventricle because of a flexible steerable and bendable distal portion (Fig. 39.3). While commercial interest in MR-compatible devices has waned, the completion of a number of positive cardiac stem cell trials has rekindled interest in MRI delivery to avoid ionizing radiation. New catheter designs are being developed incorporating features developed in MR-compatible electrophysiology ablation catheters, such as coils for tip tracking (Fig. 39.4). However, most of the device development has been performed at 1.5 T rather than 3 T, which has ramifications in spatial and temporal resolution due to the decrease signal-to-noise ratio (SNR) at 1.5 T.
INTERVENTIONAL MAGNETIC RESONANCE IMAGING TECHNIQUES
In addition to developing MR-compatible devices, a suite of imaging platforms are emerging to enable real-time interventional MR imaging of gene and stem cell delivery, using active delivery devices. Thus, in-room imaging displays and monitoring capabilities, which were originally developed for biopsy and neurointerventional procedures, are rapidly being adapted for cardiovascular applications. The inclusion of track balls and keyboards to enable full scanner operation within the scan room has now been implemented by all major MR vendors (Fig. 39.5). Feedback systems to automatically adapt the imaging planes based on catheter position or velocity are now available (33). Graphical user interfaces that allow rapid plane manipulation and table motion are becoming more common (34). Multiple approaches have been developed to fuse the signal from the catheter coils with body coil images to incorporate higher spatial resolution data from roadmaps with images acquired at a high temporal resolution to track motion. Improvements in the graphical interfaces have also been driven by the development of MR-compatible ablation systems.
However, many hurdles still remain to the widespread acceptance of interventional MRI. The implementation of methods to rapidly adjust imaging parameters “on the fly” is still limited. Although techniques, such as SENSE (35) and SMASH (36) have enabled increases in the temporal resolution of interventional MRI, even at the highest frame rates, the temporal and spatial resolutions are much lower than x-ray fluoroscopy, making precise targeting difficult in a moving heart. In addition, the high noise level of the MR suite requires specialized systems for communications within the interventionalist team.
GENE TRANSFER-GUIDED MAGNETIC RESONANCE IMAGING
The explosion of potential uses of gene therapy in clinical medicine, along with the initiation of the first human clinical trials in the United States in the 1990s, has brought forth the importance of not only interventional techniques to deliver genetic materials but also imaging methods to monitor the efficacy of gene transfection and expression. Endovascular delivery of gene products offers a method to apply high concentration of vectors in a localized region as compared to systemic intravenous administration. Besides using gene therapy to replace defective or missing genes, genes may be introduced to (a) up-regulate or inhibit expression, as in vascular endothelial growth factor (VEGF) production, to enhance angiogenesis in a stenotic vessel (37) or reduce infarct size in angina patients (38), or phospholamban inhibition of SERCA2a to enhance contractility in heart failure (39); (b) deliver genes that may produce a toxic product or cause cell suicide, as in the use of herpes simplex virus thymidine kinase/ganciclovir (HSV-tk/GCV) systems (40); or (c) act as reporter gene either to monitor the life span of a transfected cell or to image an event, such as cell differentiation that causes production of a new protein, enzyme product, or cell receptor.
There are two primary methods to transfect cells with genes. Nonviral transfection methods use either naked plasmid DNA alone or in combination with substances called transfection agents (TAs), like cationic liposomes. Viral transfection methods include retroviral, adenoviral,
adeno-associated viral (AAV), lentiviral, and herpes (simplex) viral vectors to transfer genetic material to the cell. The primary advantage of viral transfection methods is their more efficient transfection rate than nonviral transfection techniques. The negative aspect of this higher transfection efficiency is the tendency for these techniques to be immunogenic. The inability to cause stable, longterm transfection and expression can be a problem if the gene product must be expressed for the life-span of the patient, but can be an asset if the gene is only expressed for a short time period, thereby limiting the immunogenicity. In particular, AAV gene therapy has a natural tropism for cardiovascular cells, for example, smooth muscle cells and cardiomyocytes; exhibits less of an inflammatory response than adenoviral vectors; and appears to result in expression of the gene product for several months. Since drugeluting stents have lessened much of the concern about stent restenosis, gene therapy targets are now more concentrated in preventing stent graft restenosis, angiogenesis for peripheral arterial disease, and calcium transport in heart failure (41).
adeno-associated viral (AAV), lentiviral, and herpes (simplex) viral vectors to transfer genetic material to the cell. The primary advantage of viral transfection methods is their more efficient transfection rate than nonviral transfection techniques. The negative aspect of this higher transfection efficiency is the tendency for these techniques to be immunogenic. The inability to cause stable, longterm transfection and expression can be a problem if the gene product must be expressed for the life-span of the patient, but can be an asset if the gene is only expressed for a short time period, thereby limiting the immunogenicity. In particular, AAV gene therapy has a natural tropism for cardiovascular cells, for example, smooth muscle cells and cardiomyocytes; exhibits less of an inflammatory response than adenoviral vectors; and appears to result in expression of the gene product for several months. Since drugeluting stents have lessened much of the concern about stent restenosis, gene therapy targets are now more concentrated in preventing stent graft restenosis, angiogenesis for peripheral arterial disease, and calcium transport in heart failure (41).
![]() Figure 39.5. Screen capture of the Siemens prototype interactive front end (IFE) graphical interface that enables real-time scan plane manipulation and serial acquisition of up to three imaging planes. The image is reconstructed with the active injection catheter colored green for enhanced visibility. Representative pseudo long- and short-axis images are shown acquired in real-time in vivo in a canine reperfused myocardial infarction. Bookmark images (small images at bottom) facilitate rapid return to previous scan plane position using a simple drag-n-drop of the image plane into one of three image acquisition planes. (From: Kraitchman DL, Gilson WD, Lorenz CH. Stem cell therapy: MRI guidance and monitoring. J Magn Reson Imaging. 2008;27(2):299-310, with permission.) |
CONTRAST AGENTS FOR GENE TRACKING AND CELL LABELING
Because of the low background signal, labeling techniques with radionuclides for radionuclide imaging are exquisitely sensitive to an extremely low number of molecular targets. MR or CT methods would require 10- to 100-fold large amplification of these targets for detection. In addition, cellular labeling for x-ray imaging would require an iodinated contrast agent load that is incompatible with maintaining normal cellular function and viability. However, using techniques largely adapted from monoclonal antibody (MoAb) techniques of radionuclide imaging, cellular, and genetic labeling strategies for MR imaging have been developed using conventional MR contrast agents. The inherent advantage of labeling with MR contrast agents is that the cells are not exposed to radioactive species that can become especially cytotoxic when internalized.
MR contrast agents can be divided into two major classes of agents: The paramagnetic and SPIO contrast agents. Gadolinium chelates, which form the most widely used
clinically approved MR contrast agents, are in the paramagnetic class of agents. The adoption of gadolinium-based MR contrast agents for noncellular imaging is largely a result of the ability of these agents to decrease the T1 relaxation time at low doses. This decrease in T1 results in an increased signal intensity (i.e., hyperintense signal) of tissues exposed to the contrast agent using T1-weighted imaging sequences. However, because of the high toxicity of free gadolinium, chelation of gadolinium is paramount. Concerns about dechelation of the gadolinium compounds in applications for genetic and cellular labeling have largely limited the development of these agents for these purposes (42). More problematic is the reduced ability of paramagnetic compounds when internalized to affect extracellular water, and hence, the ability to alter signal intensity is greatly reduced.
clinically approved MR contrast agents, are in the paramagnetic class of agents. The adoption of gadolinium-based MR contrast agents for noncellular imaging is largely a result of the ability of these agents to decrease the T1 relaxation time at low doses. This decrease in T1 results in an increased signal intensity (i.e., hyperintense signal) of tissues exposed to the contrast agent using T1-weighted imaging sequences. However, because of the high toxicity of free gadolinium, chelation of gadolinium is paramount. Concerns about dechelation of the gadolinium compounds in applications for genetic and cellular labeling have largely limited the development of these agents for these purposes (42). More problematic is the reduced ability of paramagnetic compounds when internalized to affect extracellular water, and hence, the ability to alter signal intensity is greatly reduced.
The SPIO particles were developed shortly after the gadolinium-based contrast agents (43,44). The large magnetic moments, which are more than three orders of magnitude greater than paramagnetic-based contrast agents, of SPIO particles cause a greater effect on T2 relaxation and a smaller effect on T1 relaxation. Thus, on T2*-weighted images, SPIO particles appear hypointense and create a much larger signal change or contrast per unit of metal particle than paramagnetic contrast agents. Thus, small quantities of SPIO particles can be used for gene or cellular labeling, yet with a much larger amplification effect than paramagnetic compounds. Importantly, less agent must be internalized to create image contrast, thereby limiting cellular toxicity. Moreover, if the SPIO is degraded, the free iron that is released does not appreciably expand the native iron pool and, thus, can be degraded along normal iron recycling pathways. Most commercially available forms of SPIOs and ultrasmall SPIOs (USPIOS) have coatings to prevent particle aggregation. One of the most common USPIO coatings is dextran, which is a convenient surface for binding ligands and other functional groups for labeling. For economic reasons, an FDA-approved formulation of a dextran-coated SPIOceased product (Feridex, Bayer Healthcare), which was used extensively in preclinical stem cell labeling studies, ceased production. Recently, several groups have demonstrated the use of ferumoxytol (an FDA-approved USPIO for the treatment of iron deficiency in renal patients) in combination with protamine sulfate (an FDA-approved TA for reversing heparin therapy) to label stem cells (45,46 and 47).
IMSGING GENE DELIVERY
As stated previously, MR imaging for gene detection is largely inferior to radionuclide imaging techniques because of the adherently lower sensitivity for detecting genetic targets. Until amplification strategies to increase MR sensitivity are more fully developed, the ability to harness the high spatial resolution and interventional capabilities of MRI for cardiac gene therapies will not be recognized.
In an early attempt to apply these techniques for cardiovascular gene delivery, Du et al. (48) developed a novel MR gene delivery system that was validated in a swine model on a clinical MR scanner. A gene delivery catheter was combined with an MR imaging guidewire, and a lentiviral vector carrying green fluorescent protein (GFP) mixed with 6% gadolinium contrast agent was delivered intra-arterially and monitored in real time (Fig. 39.6). Radiofrequency (RF) heating of imaging guidewires, which is a concern of active catheter systems, has been exploited in this application by this group to enhance transfection rates (48). In a similar study to visualize gene delivery, Barbash et al. (49) used a percutaneous approach in a rat infarction model to deliver an adenoviral LacZ reporter gene mixed with gadolinium via a 22G needle. In 2013, Barbash et al. (50) performed transendocardial delivery in dogs of an AAV mixed with an iodinated contrast agent and SPIO to monitor delivery success during x-ray fluoroscopic imaging fused with previously acquire MRIs that were volume rendered.
A more sophisticated method to monitor sustained release of a gene would be to encapsulate the gene and paramagnetic contrast agent in a biodegradable sphere. Faranesh et al. (51) have developed a poly(DL-lactic-co-glycolic acid) (PLGA) microsphere containing VEGF and gadolinium diethylenetriamine pentaacetic acid (Gd-DTPA), which releases the gene and contrast agent over 6 weeks that can be noninvasively imaged as hyperenhancement on T1-weighted MRI. Microsphere systems, such as this system, enable a sustained release of the gene product over many weeks to assist with higher or sustained transfection rates, but what is unlikely is that the kinetics of Gd-DTPA and VEGF release from the microsphere are equivalent, such that absolute quantitation of gene release from MRI can be obtained using this delivery technique. Other cutting-edge techniques, which have been developed to determine cellular uptake of the gene product include binding plasmid DNA to polymers that will stabilize SPIOs (52,53) or loading micelles or nanoparticles with iron oxides and DNA (54,55) such that the transfected cells appear dark on T2*-weighted MRI.
IMAGING GENE EXPRESSION
Another area where MR delivery may be useful is in combination with ultrasonography. High-frequency ultrasound has been used to increase vascular permeability and also rupture ultrasound microbubbles (56,57). MR paramagnetic agents encapsulated in liposomes could be combined with naked DNA to enable high-resolution localization of the genes followed by enhanced transfection using highfrequency ultrasound.
Several groups have been able to develop amplification systems to visualize gene expression using MRI. In particular, Weissleder et al. (58) and Ichikawa et al. (59) have transfected tumor cells with an engineered transferrin receptor (Tfr). Monocrystalline iron oxides (MION) can be conjugated to transferrin (Tf), the natural ligand of Tfr. After systemic injection, the MION-Tf will be taken up preferentially by the tumor cells overexpressing Tfr, resulting in a hypointensity on T2*-weighted images of the tumors. In this model system, amplification has been achieved in two ways: (a) The overexpression of the receptor and (b) the ability of SPIO particles with high magnetic moments to cause large dephasing of local protons, resulting in a dramatic shortening of T2 far beyond what could be achieved by conventional gadolinium chelates.
In a similar strategy, the up-regulation of the enzyme tyrosinase in melanomas results in increased melanin production.
Like Tfr, melanin has a high affinity for iron, and gene expression can thus be probed using exogenous administration of MION (60,61). Internalization of the MION results in a bright signal in T1-weighted MRI.
Like Tfr, melanin has a high affinity for iron, and gene expression can thus be probed using exogenous administration of MION (60,61). Internalization of the MION results in a bright signal in T1-weighted MRI.
The potential exists for the development of smart MR contrast agents which either act as substrates that are cleaved by specific enzymatic activity to expose the magnetic nanoparticle or are assembled into larger polypeptides when specific enzymes or proteases are present to amplify the magnetic signal. In essence, the magnetic relaxation of the particle can be changed as a result of the increased size of the magnetic particle resulting from cross-linking, as multiple ligands are bound to multiple targets. Perez et al. (62,63,64 and 65), Bogdanov et al. (66), and Josephson et al. (67) have developed several of these enzyme-sensing particles that cluster in the presence of peroxidases. In particular, Perez et al. (65) have developed a superparamagnetic nanoparticle in this class which, in the presence of myeloperoxidase (MPO), assembles to cause decreases in spin-spin relaxation times that can be detected by MRI. Although gadolinium substrates lack the amplification of iron oxide nanoparticles, this same group has designed a nanoparticle probe with gadolinium substrates that polymerize in the presence of MPO (68). Myeloperoxidase, which is produced by activated macrophages, has been implicated
in inflammation and particularly atherosclerotic plaques. In particular, MPO activity has been detected in a rabbit atherosclerotic model as hyperenhancing regions on T1-weighted images as early as 2 hours after administration (69), that is distinct from enhancement by clinically approved Gd contrast agents. Thus, the vulnerable atherosclerotic plaque could be detected or the response of the plaque to genetic modification could be performed using this targeted MPO agent. Particles in this class can also be used as MR switches to sense the assembly and dispersal of the particles as occurring in the presence of restriction endonucleases during DNA cleavage (70).
in inflammation and particularly atherosclerotic plaques. In particular, MPO activity has been detected in a rabbit atherosclerotic model as hyperenhancing regions on T1-weighted images as early as 2 hours after administration (69), that is distinct from enhancement by clinically approved Gd contrast agents. Thus, the vulnerable atherosclerotic plaque could be detected or the response of the plaque to genetic modification could be performed using this targeted MPO agent. Particles in this class can also be used as MR switches to sense the assembly and dispersal of the particles as occurring in the presence of restriction endonucleases during DNA cleavage (70).
Another approach that may increase the MR sensitivity for clinical applications is to image a nonproton reporter probe, such as fluorine-19 (19F). Since there are almost undetectable levels of fluorine in the body, there will be a high sensitivity to detect the presence of the reporter probe using either MR spectroscopy or 19F MRI. Mizukami et al. (71) have developed a reporter probe containing gadolinium and a fluorine moiety linked by galactose; the close proximity of the gadolinium to the fluorine quenches the fluorine resonance peak. When β-galactosidase is produced by transfected cells, the reporter probe will be cleaved and the fluorine peak can now be imaged resulting as a hot spot on the MRI, which only appears where transfected cells are present. Using a different reporter probe containing 19F, Yu et al. (72) showed the ability to detect β-galactosidase activity based on a chemical shift in the fluorine peak and the trapping of ferric ions that can be detected on proton MRI.
Lanza et al. (73) and Yu et al. (74) have developed a targeted nanoparticle containing a liquid perfluorocarbon core with chelated gadolinium (Gd) complexes incorporated into the outer surface. They have overcome the amplification problem with gadolinium-based agents by two means: (a) Numerous Gd molecules can be bound to the surface of the nanoparticle and (b) antibody receptor complexes on the nanoparticle are used for targeting so that the particle is not internalized which would reduce T1 relaxivity (Fig. 39.7).
Thus, changes in T1 relaxation can be achieved in vivo. In addition, therapeutics can be incorporated into the surface of the nanoparticle for drug delivery (75). By modifying the antibody receptor complex, targeting of this agent has been performed to fibrin (i.e., thrombus) for cardiovascular applications (73,76
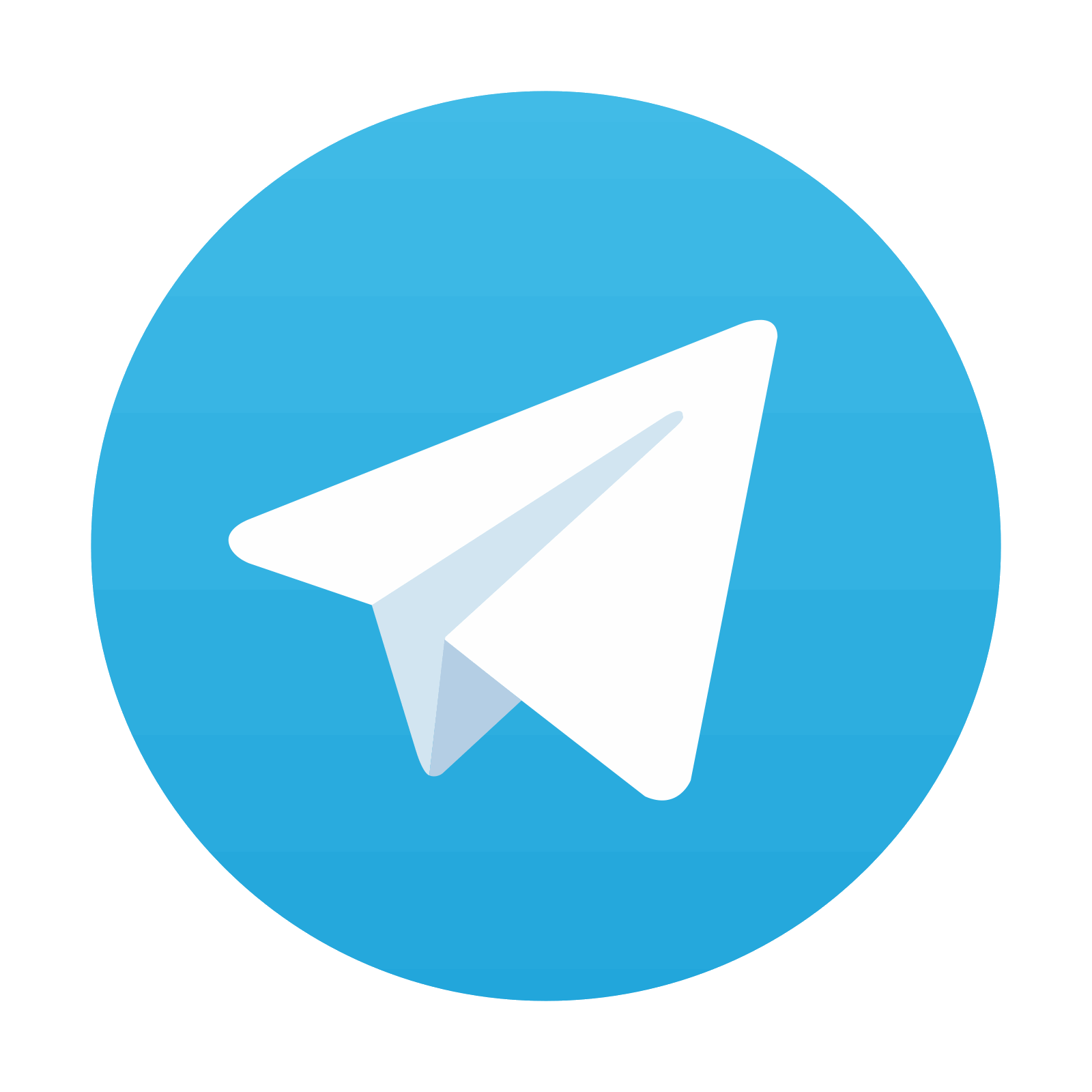
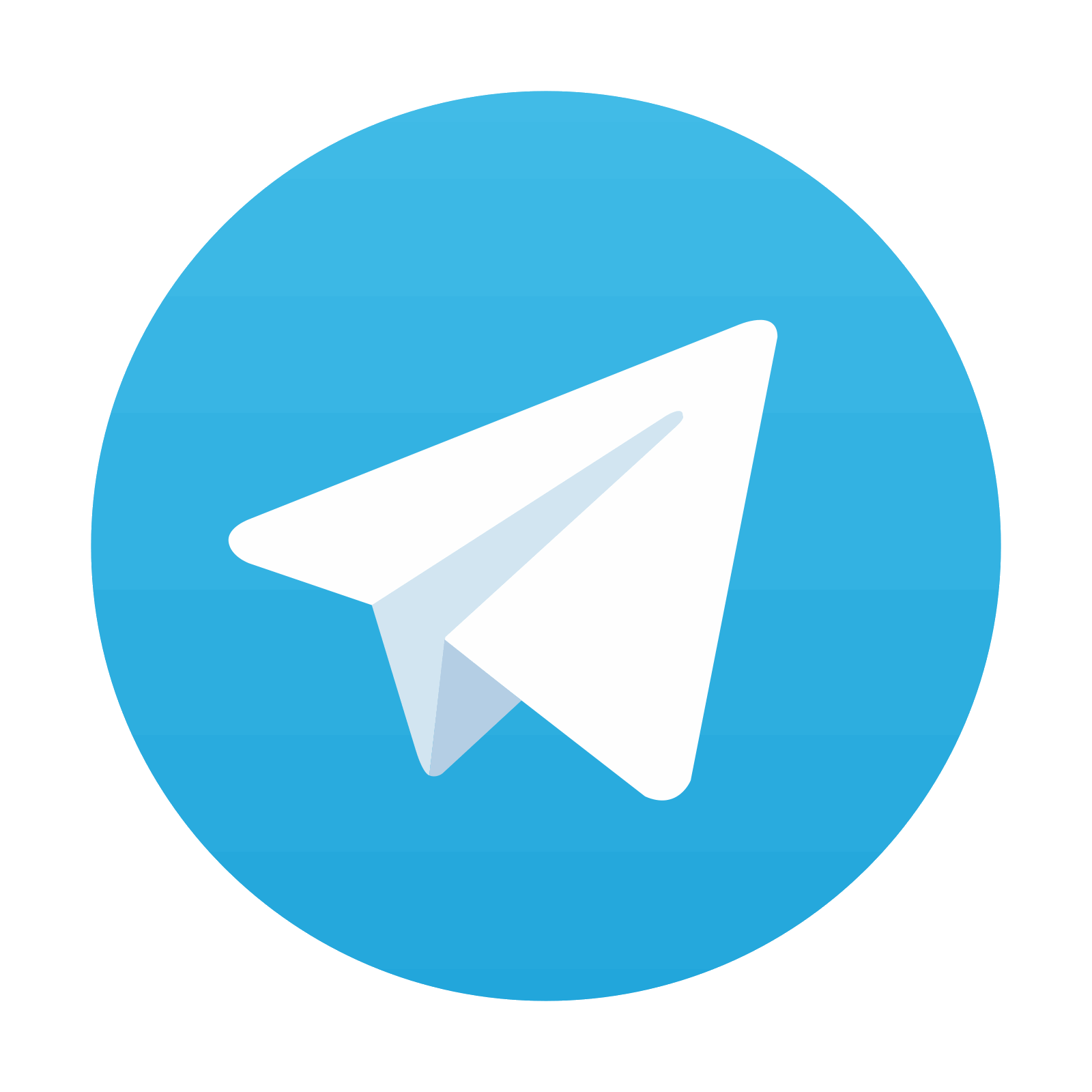
Stay updated, free articles. Join our Telegram channel
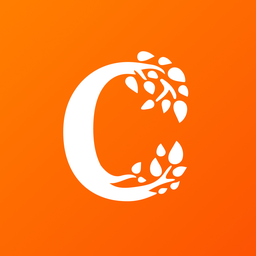
Full access? Get Clinical Tree
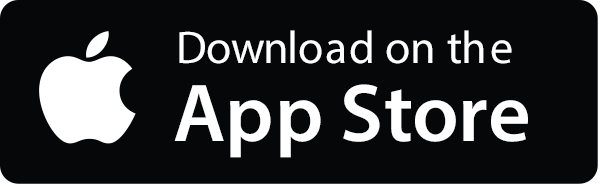
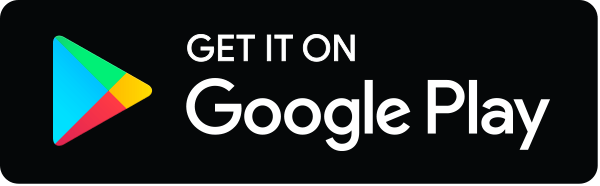