Echocardiography plays an important role in cardiac surgery by defining myocardial structure, function, and intracardiac blood flow velocities. Preoperatively, transthoracic and transesophageal echocardiography with color and spectral Doppler identify, quantify, and characterize cardiac disease and support decision making in terms of surgical timing and choices. Intraoperative transesophageal echocardiography (TEE) provides high-fidelity assessment of underlying pathophysiology, guides beating heart procedures in real time, supports surgical planning, and, allows for timely assessment of the results of surgical procedures. Furthermore, TEE can be critical in the early postoperative period to elucidate various etiologies of perioperative hemodynamic instability, allowing surgeons and intensivists to identify and manage complications accurately and efficiently. Lastly, transthoracic echocardiography (TTE) is often employed to evaluate and monitor long-term surgical results due to its noninvasive nature.
It is important that surgeons appreciate the potential and the limitations of perioperative ultrasound to provide case-specific predictors and intraoperative procedural guidance to improve surgical outcomes and facilitate incorporation of new technologies into current practice.
The goals of this chapter are to provide a framework for understanding the technique, and its indications for use. We summarize American Society of Echocardiography/Society of Cardiovascular Anesthiologists (ASE/SCA) guidelines for the surgical use of epicardial ultrasound, which can supplement or substitute for intraoperative TEE when TEE is contraindicated. Finally, we outline expectations that surgeons should have for intraoperative TEE images and interpretations.
Echocardiographic images are constructed by transmitting high-frequency sound waves into the chest from a transducer composed of piezo-electric crystals. These waves reflect off cardiac structures, and the returning signals are received by the same transducer. By knowing when the signal was sent, the speed of the sound in the tissue, and the time it takes for the reflected signal to return to the transducer, the position of the structure responsible for the reflection can be calculated. An image from these signals can thus be created. The quality of the image relies on many factors, including the media through which the sound is traveling, the orientation of the structures in relation to the ultrasound beam, and the composition of the structure. Sound travels incredibly well through water and blood, reasonably well through tissue, but poorly through air. Therefore, the media through which the sound travels will determine the strength of the returning signal. When the ultrasound beam reflects off various portions of the heart, the signal is scattered in various directions such that some never return to the transducer. For this reason, structures that are perpendicular to the ultrasound beam and reflect stronger signals back to the transducer produce images of the most accuracy. On the other hand, strong reflectors such as calcified valvular leaflets will result in a bright picture but they also inevitably create imaging artifacts. The fact that sound transmitted from a TEE transducer has less distance to travel (which means less signal lost to scatter) and mainly travels through muscle and blood (and rarely air) explains why the quality of TEE images is usually better than TTE imaging.
Echocardiographic displays include M-mode, two-dimensional (2D) and three-dimensional (3D) imaging. The M-mode echocardiogram has superior temporal resolution and can be thought of as a display of the motion of a single cut through the heart over time. It is now used primarily to quantify the timing of intracardiac events. Two-dimensional echocardiography provides a display with better spatial resolution and excellent temporal resolution. Three-dimensional echocardiography provides the best display of the spatial relationships of various structures and flow patterns, and its resolution continues to improve.
Blood flow velocities are assessed by the Doppler principle. The wavelength of sound reflecting off moving blood particles will be shorter if blood is moving toward the transducer and longer, if moving away. By quantifying this change in frequency of the ultrasound beam, one can determine the blood velocity and direction. This calculation, however, is influenced by the angle between the ultrasound beam and the blood flow such that as this angle increases, the calculated velocity is lower and less accurate. Thus the goal is to keep alignment of the beam as parallel to the blood flow as possible. As blood travels through a restrictive or stenotic region at a constant flow, its velocity will increase and the pressure gradient can be calculated from the simplified Bernoulli Equation (Δpressure = 4 × velocity2). The peak and mean pressure gradients are calculated from spectral Doppler techniques. Pulse wave (PW) Doppler has excellent spatial resolution, allowing the identification of the specific locations of flow disturbances such as obstructions or leaks. Continuous wave (CW) Doppler has excellent velocity resolution and identifies the highest velocity along the path of the ultrasound beam (which is assumed to occur at the narrowest region). Color-flow mapping displays the velocities and directions of flow determined by Doppler. This technique is valuable to identify and quantify regurgitation. However, it must be remembered that the color display of a regurgitant jet represents the velocity of the blood disturbed by the regurgitation and is not the volume of regurgitation as one would see on an angiogram. The color display of these jets is influenced by various echocardiography machine settings.
Doppler quantitation of velocities is dependent on one’s hemodynamic profiles, and since these conditions are often different during anesthesia (compared to preoperative resting state), the pressure gradients obtained by spectral Doppler and the color Doppler display of regurgitant jets may differ significantly from preoperative assessments. Thus the decision to operate should be based on preoperative values.
Finally, the echocardiographic views of the heart required to assess different pathologies as well as the necessary components of a perioperative TEE have been established by expert consensus and serve as a roadmap to understand the basic TEE.1
While TTE has some roles in the operating room, the dominant ultrasound modality for perioperative imaging is TEE. It has been in use in the operating room for over 30 years and has an excellent safety record. The incidence of complications in 7200 intraoperative TEEs performed at the Brigham and Women’s Hospital was 0.2%, and no intraoperative TEE-associated mortality was noted. The most common complication was odynophagia severe enough to warrant esophagogastroduodenoscopy (0.1%) and this was followed by acute upper gastrointestinal hemorrhage (0.03% including esophageal tear but not esophageal perforation), dental injury (0.03%), arterial desaturation due to malpositioning of endotracheal tube (0.03%), and esophageal perforation (0.01%). Unsuccessful TEE probe insertion occurred in 0.18% of cases and contraindications to TEE were noted in 0.5%.2 A smaller study has reported a higher rate of major upper gastrointestinal complications at 1.2% in patients undergoing intraoperative TEE with a significant proportion of the injuries manifesting greater than 24 hours after the procedure.3 The reported TEE complication rate in pediatric cardiac surgical patients is higher at 2.4%.4 As a reference, an older study of the complication rates in over 10,000 TEEs performed on conscious patients outside of the operating room reported an event rate similar to that of adult intraoperative patients (0.18%) however the types of complications differed and included one death.5
An important way to reduce complications, especially esophageal and gastric injury, is to prospectively recognize those patients who are at risk and avoid TEE in those with extensive esophageal and gastric diseases. Another key principle is to balance risk and benefit ratio carefully such that TEE is only performed when its benefits outweigh its risks.6 Absolute contraindications to TEE include perforated viscous, prior esophagectomy, and esophagogastrectomy. Relative contraindications to TEE include those with oropharyngeal cancer, preexisting esophageal pathologies (including varices, strictures, diverticula, esophagitis, Mallory-Weiss tears, radiation injury), and gastrointestinal pathologies (such as recent upper gastrointestinal hemorrhage, gastric ulcer or symptomatic hiatal hernia).7
Both cardiology and anesthesiology specialty and subspecialty societies have outlined recommendations for appropriate training required prior to independent performance of intraoperative TEE such as a minimum number of studies to maintain proficiency along with other benchmarks to guarantee imaging quality and practitioners’ ability to interpret findings accurately.8–12 Some surgeons have taken part in these training and certification programs, and many Cardiothoracic (CT) surgical training programs are including imaging skills-training in the curriculum.
The cardiac surgical use of ultrasound in most practices is limited to epiaortic scanning prior to aortic cannulation. Evidence is strong that routine use, coupled with alterations in surgical approach in the setting of significant atheromatous changes, reduces morbid neurological outcomes.82 The Society of Cardiovascular Anesthesiologists (SCA)/American Society of Echocardiography (ASE) Guidelines suggest measuring (1) maximal plaque height/thickness; (2) location of the maximal plaque within the ascending aorta; and (3) presence of mobile components in each of the three ascending aortic short axis segments and for the aortic arch.80,81 Figure 12-1 shows the guideline views. “Mid” is usually the aortic cross-clamp site, “distal” is usually the cannulation site.
In addition to epiaortic scanning, the epicardial probe can be utilized to supplement intraoperative TEE assessment. The ASE guidelines assist surgeons in developing expertise in obtaining meaningful images when TEE is contraindicated. Figures 12-2, 12-3, 12-4, 12-5, 12-6, 12-7, 12-8 demonstrate the recommended views, which can be obtained in a setting of a median sternotomy incision. These ASE views serve as a general suggestion to help develop a user-dependent approach that is tailored to best suit each surgeon’s individual practice.
FIGURE 12-4
Epicardial left ventricular (LV) basal SAX view (TTE modified parasternal mitral valve basal SAX equivalent). With the transducer orientation marker facing the left shoulder, place the transducer over the body of the right ventricle (RV). View RV with tricuspid valve and LV with mitral anterior (AL) and posterior (PL) leaflets in short axis.

It is suggested that surgeons take a few moments when they have the ultrasound probe in the surgical field to practice obtaining these standard ASE images. In the situation of a critical need for intraoperative ultrasound imaging when TEE is contraindicated (noted above in the section Safety of Intraoperative Transesophageal Echocardiography), a standard epicardial probe can be used if there is a full sternotomy. If there is a minimally invasive incision, placing the TEE probe in a sterile sheath filled with ultrasound gel can also provide excellent epicardial images of all structures from almost any small incision, which allows access to the pericardial space. However, in order for this to be most successful, the surgeon should practice along with the assistance of the echocardiographer as a team to obtain useful views—with the echocardiographer manipulating the controls and the surgeon manipulating the TEE probe over the heart. The same locations used in the ASE epicardial guidelines can be used, but relevant views can be obtained from many locations in the pericardial space.
Once the heart is closed and begins to beat at the end of cardiopulmonary bypass, pockets of air are often present in the left heart and the ascending aorta. Air can change locations quickly. The ability to precisely locate and evacuate air decreases the time from cross-clamp removal to successful weaning from cardiopulmonary bypass and decreases short- and long-term morbidity. Thus it behooves the surgeon to understand how ultrasound imaging can guide this process. The specific views most likely to eludicate air pockets are the left atrial dome view, left and right superior pulmonary vein views, left atrial appendage view, and LV apex and LV septal wall views. Figure 12-9 shows all of these views from the TEE window, but the information can also be obtained by the surgeon using an epicardial probe in the event that TEE is not available.
FIGURE 12-9
Diagnosis of intracardiac air at the end of cardiopulmonary bypass—common air locations. The “A” panels show the anatomy without air, and the “B” panels show the same views with air present. Panel 1 is 120 Mid esophageal long axis (120°) with air in the LVOT, Panel 2 shows dome of the LA with air behind the aortic valve in the LA, Panel 3 is a view of the L superior pulmonary vein, and Panel 4 is the right superior pulmonary vein with two comet tail artifacts indicating air in both branches of the vein.

Echocardiography diagnoses the presence of ischemic heart disease by demonstrating resting LV wall motion abnormalities or inducing new regional wall dysfunction with stress modalities.
The ASE recommends a 16-segment model for the assessment and description of segmental LV wall motion.13 This model divides the LV basal and mid-ventricular short axis views in a clockwise fashion into anterior, anterolateral, lateral, inferolateral, inferior, inferoseptal and anteroseptal segments. The apex is divided into anterior, lateral, inferior and septal segments (Fig. 12-10). A 17-segment model also exists where there is an additional apical cap to assess myocardial perfusion.
FIGURE 12-10
Echocardiography segments of the left ventricle. Orientation of the apical four-chamber, apical two chamber and apical long axis views in relation to the bull’s eye display of the LV segments. (Reproduced with permission from Lang RM, Badano LP, Mor-Avi V, et al: Recommendations for cardiac chamber quantification by echocardiography in adults: an update from the American Society of Echocardiography and the European Association of Cardiovascular Imaging, J Am Soc Echocardiogr 2015 Jan;28(1):1-39.)

To assess wall motion, each segment is evaluated in multiple views and reported to be (1) normal or hyperkinetic (normal or enhanced wall thickening), (2) hypokinetic (reduced thickening), (3) akinetic (absent or negligible thickening), or (4) dyskinetic (systolic thinning or bulging).13
Resting regional hypokinesis is indicative of ischemia or infarction in a specific coronary artery distribution that supplies the particular segment. Myocardial infarction with scar appears as a thin, highly reflective or bright, akinetic segment. An aneurysm is a thin dyskinetic segment that has a distorted diastolic shape and bulges outward in systole.
Stress echocardiography diagnoses and localizes obstructive coronary artery disease (CAD) and is performed with exercise or pharmacological agents. Exercise with supine bicycle or treadmill is physiologic and always preferred to pharmacological stress testing. Patients unable to exercise may be stressed with inotropic drugs (dobutamine), vasodilator agents (adenosine or dipyridamole) or a combination of these. Stress echocardiography has a specificity of 76% and sensitivity of 88%, which are comparable to nuclear stress modalities.14
In a normal stress test, there is a hyperdynamic response with an increase in left ventricular ejection fraction (LVEF), decrease in LV cavity size, and absence of new regional wall motion abnormalities. A positive stress test demonstrates new inducible wall motion abnormality in the ischemic segment with stress. Decreases in global LVEF and LV dilatation suggest the presence of severe obstructive CAD such as multivessel CAD or severe left main stenosis.15
Intravenous echocardiographic contrast agents can be used to enhance endocardial definition, hence increasing the sensitivity of stress echocardiography for the diagnosis of ischemic heart disease.
Low-dose dobutamine stress echocardiography is utilized to identify viable myocardium that is stunned or hibernating, and has the potential to recover after revascularization. Viable segments are hypokinetic at rest and typically demonstrate a “biphasic response” with an initial improvement of myocardial contractility with low-dose dobutamine infusion (2.5 to 10 mcg/kg/min). However, at higher doses of dobutamine (20 to 40 mcg/kg/min), the segmental wall motion deteriorates and appears similar or worse than at rest. There is a strong association between myocardial viability on noninvasive testing and improved survival after revascularization in patients with chronic CAD and LV dysfunction.16 An area that fails to augment is indicative of a nonviable segment or scar.
Patients with acute myocardial infarction who develop hemodynamic instability should have an echocardiogram as soon as possible to differentiate mechanical complications that require urgent surgical intervention, from cardiogenic shock secondary to primary ‘pump’ failure.
Rupture of the LV free wall is the second leading cause of in-hospital death, after cardiogenic shock in the setting of an acute myocardial infarction. It is usually associated with a first transmural (usually large anterior) infarct, but not infrequently it can also occur in a small area of lateral necrosis. Acute rupture is catastrophic and rapidly fatal, with death ensuing from cardiac tamponade and pulseless electrical activity. Subacute rupture is a gradual and incomplete rupture of the infarcted area with slow or repetitive bleeding into the pericardial sac causing hemopericardium, which typically occurs through small intramyocardial communications.17 This leads to a moderate to large pericardial effusion, with or without features of tamponade. Often thrombus forms in the myocardial channels that communicate with the pericardial space, which prevents continued rupture. Thrombus in the pericardial space can be observed as a mass of echoes.
A postmyocardial infarction (post-MI) LV pseudoaneurysm is a rupture of the ventricular free wall contained by local pericardial adhesions or organized thrombus. Echocardiography identifies a narrow neck of the pseudoaneurysm and often spectral and color Doppler imaging shows flow through the communication between the LV and the pericardium.
Post myocardial infarction VSRs are uncommon but associated are with high mortality and usually occur in a large transmural, index infarct. Approximately 50 to 66% of postinfarction VSRs occur in anterior infarctions with the rest seen in inferior infarctions.18,19 A VSR after an anterior MI most often occurs in the distal 1/3 of the septum whereas an inferior MI is associated with rupture of the basal inferior septum. Off-axis 2D and color Doppler imaging are useful, especially to demonstrate the RV exit site (Fig. 12-11). The size of the defect and the pressure difference between the ventricles determine the amount of shunting, which in turns influences mortality. Three-dimensional echocardiography can visualize the defect en face to demonstrate the precise location of the rupture and provide size measurements. This can be helpful in determining the suitability of percutaneous device versus surgical closure.20
FIGURE 12-11
Post MI ventricular septal rupture. (A) Transthoracic echocardiographic off axis apical four-chamber view displaying the RV exit site of the VSR at the mid-septum. (B) Color Doppler displaying left to right shunting across the VSR in systole. RV = right ventricle, VSR = ventricular septal rupture.

Acute mitral regurgitation (MR) in the setting of infarction is caused by papillary muscle infarction or rupture. It is usually associated with an inferior infarct as the posteromedial papillary muscle has a single blood supply from the posterior descending artery, whereas the anterolateral papillary muscle has dual blood supply from the left anterior descending artery and the left circumflex artery. Two-dimensional echocardiography demonstrates a flail segment of the mitral valve. The ruptured head of the papillary muscle or corresponding chords may be seen moving randomly within the LV cavity (Fig. 12-12).
Color Doppler can underestimate the severity of acute MR. Due to rapid equalization of left atrial (LA) and LV pressures, coupled with tachycardia and the usually small LA size, the MR jet area can be of short duration and appear deceptively small. Assessment by vena contracta width remains reliable in this setting. Pulmonary venous systolic flow reversal is a feature which supports the presence of acute severe MR.21 Assessment with TEE can provide a more definitive diagnosis.
Echocardiography is a valuable diagnostic test for RV infarction. The most common features of RV infarction include inferior MI and hemodynamic compromise, often associated with jugular distension and absence of pulmonary edema. RV involvement is associated with higher mortality, arrhythmias, shock and mechanical complications.22 Echocardiographic signs of RV infarction include RV dilatation and decreased RV free wall function. Impaired function is demonstrated by reduced tricuspid annular plane systolic excursion (TAPSE < 17 mm), and decreased tricuspid annular systolic velocity measured by Doppler tissue imaging (S′ velocity < 9.5 cm/s).13,23 Signs of elevated right atrial (RA) pressure include a dilated inferior vena cava (IVC) that does not collapse >50% on inspiration or bowing of the interatrial septum towards the left.
Echocardiography of the mitral valve (MV) includes evaluation of the valvular leaflets, mechanism and grading of severity of mitral stenosis (MS) and mitral regurgitation (MR), and assessment of the effects on the LA and LV. Specifically this includes 2D imaging of leaflet thickness, mobility, coaptation geometry and area, LA size, LV size and function, spectral and color Doppler, valvular gradients, valvular area, and RV systolic pressure (RVSP).
MR can be classified into organic (primary) or functional (secondary). Organic MR is caused by intrinsic valvular disease whereas functional MR occurs as a result of regional or global left ventricular remodeling.
The most common cause of MR in the industrialized world is degenerative MV disease. The spectrum of conditions ranges from fibroelastic deficiency causing isolated prolapsed segments due to chordal rupture, to extensive myxomatous disease affecting all segments of the valvular leaflets. The former is most often seen in the older population whilst the latter in younger patients.
Mitral valve prolapse (MVP) is defined by displacement of the body of the leaflets by more than 2 mm behind the annular plane, measured in a long axis view (parasternal or apical views). Due to the saddle shape of the normal mitral annulus with its lowest points anteriorly and posteriorly, assessment in the apical four-chamber view which depicts the medial and lateral MV annulus is not recommended as this can lead to a false diagnosis of MVP.24
Flail leaflet is a common sequel of myxomatous disease and occurs due to ruptured chordae. The posterior leaflet (usually middle or P2 scallop) is predominantly affected, resulting in anteriorly directed MR. On echocardiography, the flail portion of the leaflet will exhibit excessive motion and the leaflet tip is displaced into the LA beyond the mitral annular plane (Fig. 12-13).
Other less common causes of primary MR include rheumatic heart disease, infective endocarditis, mitral valve cleft (in conjunction with a partial atrioventricular septal defect, or rarely in isolation), connective tissue diseases, and radiation induced heart disease. Of note, rheumatic mitral regurgitation is caused by commissural thickening, leaflet motion restriction, and loss of central coaptation. This is often accompanied by a degree of stenosis.
Acute MR can be assessed by echocardiography and occurs due to disruption of the MV complex. Leaflet destruction or perforation occurs in infective endocarditis. Chordal rupture can be associated with endocarditis, but is more often seen in degenerative mitral valve disease. Papillary muscle dysfunction (rupture or ischemia) occurs with MI (usually the inferior territory). Due to the acute nature of the MR, the LV and LA sizes are often normal. LV systolic function is normal or hyperdynamic, except when the acute MR is due to myocardial ischemia.
In functional (or secondary) MR, the mitral valve leaflets and subvalvular apparatus are structurally normal. The MR occurs as a result of LV remodeling from previous infarction or nonischemic cardiomyopathy. Regional or global LV dilatation and remodeling leads to displacement of the papillary muscles, stretching of chordae and subsequent leaflet tethering. The coaptation zone of the mitral valve leaflets is displaced apically and there is incomplete closure of the mitral valve. Dilation of the mitral annulus also contributes to functional MR (Fig. 12-14).
FIGURE 12-14
Incomplete closure of the mitral valve on apical four-chamber TTE. (A) There is global left ventricular dilation resulting in displaced papillary muscles. The mitral valve leaflets are pulled apically resulting in incomplete closure of the mitral valve. (B) Large central jet of mitral regurgitation.

Regional infarction (particularly of the inferoposterior territory) and subsequent local remodeling leads to unbalanced tethering forces that cause asymmetric closure of the valvular leaflets. In such cases, the anterior leaflet tip may appear to prolapse relative to the tethered posterior leaflet, resulting in a posteriorly directed MR jet. In patients who develop diffuse LV dysfunction and global LV dilation either from ischemic or nonischemic causes, the papillary muscle displacement is more symmetric and the resultant MR is centrally directed.
Carpentier has developed a functional classification to reflect the pathological changes based on leaflet motion that caused MR: normal, excessive, restricted diastolic and restricted systolic motion.25 (Table 12-1) Both TTE and TEE can be used to identify these patterns.
Organic MR | Functional MR | ||
---|---|---|---|
Normal leaflet motion (Type I) | Excessive leaflet motion (Type II) | Restricted diastolic leaflet motion (Type IIIa) | Restricted systolic leaflet motion (Type IIIb) |
|
|
|
|
The timing for intervention in MR is largely driven by symptoms and the impact of the volume load on LV size and function. Thus, accurate assessment of the severity of MR and the associated hemodynamic consequences is critical. Typical methods and supporting parameters are shown in Table 12-2. Assessment of severity requires the integration of both qualitative and quantitative methods to minimize the effect of technical or measurement errors. For example, the severity of MR by color Doppler can be influenced by blood pressure, and MR can appear less severe during TEE compared to TTE, especially when lowered blood pressure is secondary to sedation or general anesthesia.
Semi-quantitative
|
Quantitative: proximal isovelocity surface area (PISA) method
|
Supportive
|
Assessment of the regurgitant jet size and extent into the LA by color flow Doppler is the easiest and most common way to qualitatively assess the severity of MR. Generally speaking, an MR jet area >40% of the LA is consistent with severe MR.21 However, this method is dependent on multiple hemodynamic and technical factors. For example, the driving blood pressure, machine settings such as gain or velocity scale, and regurgitant jet direction will all influence the appearance of the regurgitant jet area. When it comes to regurgitant jet direction, if MR jets are directed towards the wall of the LA (ie, eccentric MR jets), then jet energy (velocity) is lost and eccentric MR jets would appear smaller on screen when compared to central MR jets despite similar regurgitant volumes.26 Color flow should be used to diagnose presence of MR, but quantitative measures should be utilized when more than a small central MR jet is present to avoid this problem.
The vena contracta is the region of the jet as it exits the regurgitant orifice. When quantified it is an indirect measure of the effective regurgitant orifice area (EROA) and this measurement is less subject to the factors affecting display of the color jet area. The VC is typically measured at the narrowest portion of the MR jet just beyond the coaptation line (Fig. 12-15). When measured as a linear dimension, it is assumed that the regurgitant orifice area is circular; however VC has been found to often be elliptical in functional MR. A VC of >7 mm is consistent with severe MR. Intermediate values (3 to 7 mm) need confirmation by other quantitative methods.
Quantitative parameters that assess MR severity include EROA, regurgitant volume (R Vol) and regurgitant fraction. These can be calculated by the proximal isovelocity surface area (PISA) method with echocardiography.
This method relies on the fact that the regurgitant flow at the regurgitant orifice is equal to the product of the cross-section area (CSA = 2πr2) of the flow and the peak velocity of the flow (peak MR vel). By adjusting the Nyquist limit (the velocity at which the signal is aliased) towards the direction of the regurgitant jet, a flow convergence zone in the LV with radius r can be depicted and measured.
Assuming that the flow converges onto the regurgitant orifice in an axis-symmetric fashion, the flow of this zone can be identified as 2πr2 × aliasing velocity (Va) and will also be equal to the flow through the valve. This can be expressed as the product of the EROA and the peak velocity of the MR CW Doppler profile. Rearranging this continuity of flow relation yields:
Since stroke volume is quantified as the product of the cross-sectional area and the velocity time integral of the CW MR velocity profile (VTIMR), the regurgitant volume (R Vol) is expressed as
Qualitatively, the presence of flow convergence on TTE at a Nyquist limit of 50 to 60 cm/s should alert the surgeon to the presence of significant MR and prompt an assessment by quantitative method. The two-dimensional isovelocity surface area (2D PISA) approach is performed in either the four- or three-chamber view (Fig. 12-16).
FIGURE 12-16
PISA method to quantify the severity of MR. (A) Measurement of the radius of the flow convergence zone (double-headed arrow). The Nyquist limit baseline is shifted in the direction of the MR jet (red arrow). (B) The maximum velocity and VTI of the MR jet is measured from the continuous wave Doppler jet profile.

The regurgitant fraction can be calculated from stroke volume calculations if there is no aortic regurgitation present. The regurgitant fraction is the ratio of the volume of MR to the mitral inflow stroke volume. The volume of MR is the difference between the mitral inflow stroke volume and the aortic outflow stroke volume. The stroke volumes are calculated as the product of cross-sectional area (CSA) and velocity time integral (VTI) of flow. Thus, the volume of MR is the difference between (1) the product of the cross-sectional area of the mitral annulus and the VTI at the mitral annulus and (2) the product of the cross-sectional area of the left ventricular outflow tract (LVOT) and the VTI at the LVOT.
Direct measurement of the EROA by 3D planimetry of the vena contracta is feasible, does not require geometric or flow assumptions, and may improve the accuracy of MR grading when compared to the 2D PISA method.27 However, due to technical limitations such as spatial resolution, 3D color Doppler to date is used only in TEE and mainly for research applications.
Adjunctive parameters help consolidate determination of the severity of MR when there is discrepancy between the quantified degree of MR and the clinical context.
Systolic reversal of flow in pulmonary veins as assessed by pulse wave Doppler is a reliable marker of severe MR. Blunting alone of systolic flow can accompany mitral regurgitation of increasing severity, but this phenomenon also occurs in atrial fibrillation, increasing age and diastolic dysfunction or other causes of elevated left atrial pressure. Therefore blunting of the pulmonary venous systolic flow lacks specificity for severe MR.
MR of increasing severity leads to increased transmitral forward flow in early diastole, reflected by an elevated E velocity. In the absence of mitral stenosis (MS), a peak E velocity of >1.5 m/s is suggestive of severe MR, whereas the presence of a dominant A wave excludes it. This feature however lacks specificity since anything that raises LA pressure can lead to increased E velocity.
Severe MR produces a dense CW Doppler signal intensity. The CW display of MR velocity can demonstrate a “cut-off” sign, appearing more triangular than parabolic (reflecting the effect of the MR on LA pressure). It may be difficult to capture the full envelope of the MR jet in eccentrically directed MR despite the relatively dense velocity signal.
The quantification of severity in functional MR can be difficult. In patients with functional MR, adverse outcomes are associated with a smaller calculated EROA compared to organic MR. Functional MR will progress due to associated progressive LV systolic dysfunction from the underlying myopathic process and adverse remodeling. There is also an underestimation of EROA by the 2D echocardiography derived flow convergence method due to the fact that the regurgitant orifice may not be circular. Hence, the threshold to define severe functional MR is lower than that used for organic MR. An EROA ≥ 40 mm2 or R Vol ≥ 60 mL indicates severe organic MR while an EROA ≥ 20 mm2 or R Vol ≥ 30 mL identifies patients at risk of increased cardiovascular events with functional MR (Table 12-3).28
|
In order to maintain forward stroke volume in chronic significant MR, the LV end diastolic volume increases in response to volume overload. This, in turn, leads to an increased LVEF. A “normal” LVEF in MR is approximately 70%. Over time, as LV contractility declines, the LV end systolic volume also increases, leading to a reduction in LVEF. Once the LVEF declines below 60%, or when the LV end systolic dimension is >40 mm, this suggests the onset of LV dysfunction, even though the LVEF is still in the normal range. LA dilation also occurs with MR of significant severity (except in acute MR), and therefore the presence of LA dilation suggests chronicity.
In primary MR, negative prognostic features include presence of symptoms, onset of LV dysfunction, pulmonary hypertension (pulmonary artery systolic pressure >50 mm Hg) and onset of new atrial fibrillation.29,30 Thus these findings are triggers for mitral valve surgery in the 2014 American Heart Association (AHA)/American College of Cardiology (ACC) guidelines (Fig. 12-17).
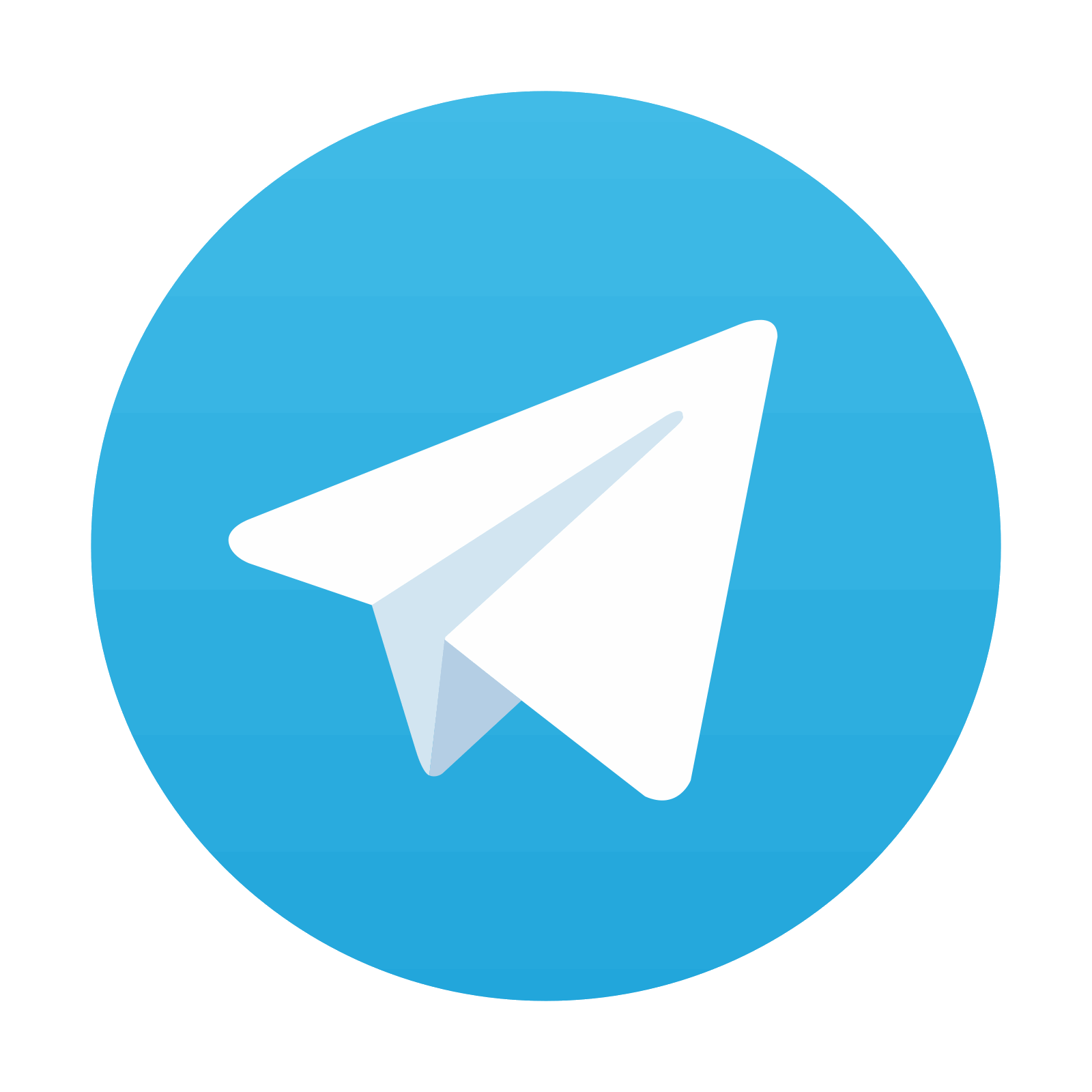
Stay updated, free articles. Join our Telegram channel
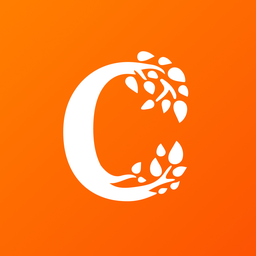
Full access? Get Clinical Tree
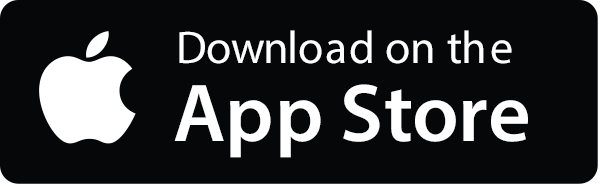
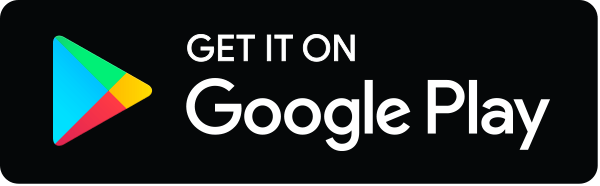