Echocardiography
Edwin Ho
Cynthia Taub
INTRODUCTION
Echocardiography is an imaging modality that utilizes ultrasound to visualize cardiac structure and function. It is the most commonly used imaging technique in cardiology because of the detailed information that can be obtained in a relatively short time, its widespread availability and portability, minimal inconvenience and discomfort for patients, and the lack of ionizing radiation exposure. Over time, the technology and techniques used for echocardiography have evolved to provide more detailed anatomic and cardiac functional assessment than ever before. In addition to two-dimensional and Doppler-based imaging, three-dimensional imaging and strain echocardiography are now integrated into routine clinical practice in certain settings.
Transthoracic echocardiography (TTE) involves image acquisition from outside the body through well-defined standard imaging windows and planes.1 Transesophageal echocardiography (TEE) provides complementary information by allowing greater spatial resolution in the assessment of certain cardiac structures, such as the atrioventricular valves, because of the proximity of the imaging probe to these structures within the heart. Image acquisition, in this case, involves a transducer that is introduced into the esophagus and stomach through the mouth or nasal cavity.2,3
INDICATIONS
Transthoracic Imaging
TTE is the most common echocardiographic modality and has a large number of indications. These can be categorized on the basis of the cardiac structure of interest and associated disease states: left ventricle, right ventricle, atria and the interatrial septum, valves, pericardium, aortic root, aorta, and pulmonary trunk.4,5 Alternatively, indications can also be categorized on the basis of symptoms and the potential cardiac causes within the differential diagnosis, such as chest pain, exertional dyspnea, or syncope. Special indications also include titration of device therapy, such as temporary or long-term left ventricular assist devices or for guidance of minimally invasive cardiac procedures.6,7,8
Transesophageal Imaging
TEE provides greater spatial resolution of the cardiac structures deeper within the thoracic cavity, because of closer proximity to the esophagus compared to the chest wall. Common indications include more detailed assessment of native or prosthetic valvular structure and function, evaluation for intracardiac masses, atrial and atrial appendage pathology including masses and thrombi, and assessment of the interatrial septum for atrial septal defects or a patent foramen ovale.9,10,11,12,13 This technique is also being used more and more to guide transcatheter cardiac procedures, such as those performed for valve repair or replacement.7
Stress Echocardiography
Stress echocardiography is most commonly indicated to assess for inducible ischemia in the setting of suspected or known ischemic heart disease.14,15 This can be performed using exercise stress with either a treadmill or supine bicycle according to standard exercise protocols. Alternatively, pharmacologic stressors such as dobutamine can also be used when exercise cannot be performed to reach a target heart rate. Dobutamine stress echocardiography can also be used to assess for myocardial viability when it is unclear if there is viable hibernating myocardium within a coronary territory.
In addition to coronary artery disease, stress echocardiography can provide clinically relevant information in nonischemic heart disease.16 This is often used to evaluate valvular heart disease such as mitral stenosis, and exercise-induced diastolic dysfunction as well. Exercise stress echocardiography can objectively reveal exercise tolerance and assess hemodynamic changes with exercise in patients with hypertrophic cardiomyopathy, pulmonary hypertension, and nonischemic cardiomyopathy. Low-dose dobutamine stress echocardiography plays a role in clarifying the degree of aortic stenosis in low-flow, low-gradient aortic stenosis with decreased left ventricular ejection fraction (EF).17,18,19 Lastly, exercise capacity and myocardial performance augmentation may also be relevant in the diagnosis and risk stratification of cardiomyopathy such as peripartum cardiomyopathy.
Point-of-Care Ultrasound
Point-of-care ultrasound (POCUS) is becoming more commonly used with the miniaturization of echocardiography hardware and advancements in portable computing ability. It is used to provide additional diagnostic information or to monitor response to therapy during the assessment of acute illness, such as in the emergency department or intensive care unit. Examples of the utility of POCUS include assessment of volume status, pericardial or pleural effusions, evaluation of
left ventricular function and wall motion, assessment for right ventricular dysfunction associated with pulmonary embolism, or screening for mechanical complications of acute myocardial infarction.20
left ventricular function and wall motion, assessment for right ventricular dysfunction associated with pulmonary embolism, or screening for mechanical complications of acute myocardial infarction.20
ANATOMIC CONSIDERATIONS
Acoustic Windows
Transthoracic
Imaging windows and views for TTE have been well defined and described (Figure 34.1).1 Study protocols often begin in the left lateral decubitus position with parasternal images, taken from the anterior chest just lateral to the left side of the sternum. This imaging window is found in the third intercostal space, but higher or lower windows can be used in certain situations. Next, apical images are taken by moving the transducer to the apex of the heart, typically near the left anterior axillary line in the fifth or sixth intercostal space. Subcostal images are taken from the upper abdomen just inferior to the xiphoid process and angulating the transducer head cranially, with the patient supine and abdominal muscles relaxed. The suprasternal window is located above the sternal notch at the lower part of the anterior neck, with the transducer head angled caudally. Additional imaging windows can be used in special circumstances. For example, a right parasternal window on the right side of the sternum can provide better visualization of the proximal ascending aorta.
In each acoustic window, the imaging plane can be adjusted to visualize specific cardiac structures by manual rotation of the transducer, or electronically through the control console if using a three-dimensional matrix transducer. Standard imaging planes are outlined in Figure 34.1.
Transesophageal
Typical imaging windows for TEE have also been well described (Figure 34.2B).2 Most images are taken from the mid-esophageal position, when the transducer head is located directly behind the left atrium and faces the apex of the heart. From this position, cardiac structures can be seen in different planes by rotating the imaging plane angle on a three-dimensional matrix transducer, adjustment of the probe deeper or shallower in the esophagus, rotating the entire probe, and by gentle adjustments of the distal probe position through ante-, retro-, left-, and right flexion (Figure 34.2A). Additional images are also taken from the transgastric window, when the transducer head is passed through the gastroesophageal junction into the stomach. Modified windows higher or lower in the esophagus can also be used for more detailed assessment of specific cardiac structures.
FUNDAMENTALS OF ECHOCARDIOGRAPHY
Physical Principles, Instrumentation, and Basic Ultrasound Physics
Echocardiography is based on the physical principles of ultrasound imaging. Piezoelectric crystals are arranged in a linear or matrix orientation inside a transducer and emit high-frequency (1-10 MHz) sound waves. These waves travel into the body through direct contact, often also assisted using viscous gel that facilitates sound wave transmission. When these sound waves travel through the body, they interact with tissues and reflect back toward the transducer in a variable manner depending on the depth of the structure and reflecting characteristics. Once received at the transducer, the reflected signal is processed into visual images to allow for interpretation. This is possible because the speed of sound can be used to calculate the depth of a structure that is reflecting the signal. The intensity of a reflection is also processed when an image is created. Because sound waves travel poorly through air and bone, the lungs and bony structures can cause significant shadowing where structures cannot be seen.
The axial spatial resolution of a single ultrasound beam is related to the wavelength of the emitted waves. Wavelength (λ) is inversely proportional to frequency (f) when the velocity of ultrasound propagation (c) is constant. In soft tissues, c ≈ 1540 m/s, and therefore f ≈ c/λ. Accordingly, higher frequency sound waves will produce shorter wavelengths and better resolution. Unfortunately, higher frequency waves are not able to penetrate tissue as effectively and may not be usable if the target structure is deeper. Harmonic imaging (as opposed to fundamental imaging) is based on the principle that tissue which is insonified by ultrasound beams can vibrate at twice the frequency of the source ultrasound waves. These second-order harmonics are received by the transducer and greatly increase signal-to-noise ratio because they are less prone to acoustic interference.
Overall image quality depends on several factors. Lateral resolution is affected by the number of scan lines used to generate an image frame, and is often maximized when the scan sector width is minimized. Ultrasound beams also vary in width along their path depending on the timing of the piezoelectric elements that emit the beam. This narrowest point can be adjusted and defines a depth where lateral resolution is further optimized.
Because sequential frames of a two-dimensional ultrasound image are created by repeated pulses that create scan lines, the temporal resolution, or time required between one frame and the next, will vary depending on the number of pulses needed (related to the scan sector width) and time needed for reflected pulses to be received (related to the scan sector depth). Narrower and shallower scan sectors will increase the temporal resolution, allowing fast-moving cardiac structures to be better visualized.
M-Mode, Two-Dimensional, and Three-Dimensional Imaging
Early generations of echocardiography equipment transmitted a single sound beam only, resulting in a single scan line. This could be displayed in “A-mode” (amplitude), where amplitude of reflections was depicted as spikes from a baseline. “B-mode” maps the amplitude of reflections into a grayscale depiction and represents the ultrasound images we are familiar with currently (Figure 34.3A). Two-dimensional B-mode imaging with a wider field of view than a single scan line is possible because modern transducers contain numerous piezoelectric elements that will electronically steer the sound beam to sweep the scan line and reconstruct an image based on the signal received from each scan line position. “M-mode” (motion) maintains a single scan line only, but maps the reflected signal using a grayscale map over time on the horizontal axis (Figure 34.3B). The advantage of M-mode imaging is its superior temporal resolution.
Modern ultrasound equipment uses transducers containing a large number of emitting and receiving elements that emit and receive ultrasound pulses in an ordered sequence, rather than in a continuous manner. This sequential pulse emission repeats over and over to create moving images, and, therefore, the maximum temporal resolution has an upper limit. The speed of sound in tissue and desired depth of visualization both affect the “pulse repetition frequency (PRF),” or pulse generation rate, because adequate time is required to receive reflected sound waves.
Three-dimensional echocardiography has been made possible because of the modification of transducer crystals into a matrix array (gridlike arrangement) so that sequential pulse emission can occur in a two-dimensional plane instead of only in a linear manner (Figure 34.3C,D). Temporal resolution is described as a volume rate because three-dimensional ultrasound volumes are generated instead of two-dimensional image frames.
Doppler Ultrasound
Doppler echocardiography is based on the Doppler principle:
, where f = Doppler shift frequency, v = velocity of target, f0 = frequency of transmitted ultrasound beam, θ = angle between the ultrasound beam and direction of flow, and c is velocity of ultrasound in blood. In other words, motion will modify the perceived frequency of a sound wave from that object. This is due to compression of the waves if the object is moving toward the receiver and stretching if moving away. In echocardiography, this principle is commonly applied to sound reflections coming from moving red blood cells. The observed frequency shift can be converted into a velocity using the Doppler equation, and this information can then be displayed two-dimensionally using a color map (Figure 34.4A) or as a plot of velocity versus time at one specific sampling location (Figure 34.4B) or along an entire line of interrogation (Figure 34.4C).

Pulsed-Wave Doppler Echocardiography
Pulsed-wave Doppler utilizes separated pulses of ultrasound waves with an adequate time window to receive reflections (PRF) to define velocity at a specified depth and location within the heart. This is typically used to assess blood velocity at the sample volume (location of interest) defined by the operator. Because the PRF has an upper limit based on the depth of the sample volume, the maximum velocity that can be interrogated is also limited. This is due to the fact that the degree of Doppler frequency shift is greater for faster objects and needs to be sampled faster than the PRF may allow. The Nyquist limit is the maximum velocity that can be accurately determined using pulsed-wave Doppler, and is typically around 1.5 m/s. Beyond this velocity, the specific velocity of the reflection is uncertain.
Continuous-Wave Doppler echocardiography
Continuous-wave Doppler is performed by continuous emission and reception of ultrasound waves along a single line. Depth of reflections cannot be determined in this case because continuous ultrasound emission means the timing of a received reflected signal cannot be used to determine the depth of origin. However, this technique removes the Nyquist limit restriction of pulsed-wave Doppler, allowing essentially the maximum velocity across a path to be determined.
Color Doppler Echocardiography
Color Doppler imaging is based on the same principles of pulsed-wave Doppler, except that an entire region of interest is assessed instead of a specific small sample volume. This means the temporal resolution must be lower. Average velocities and direction of movement are displayed using a color map superimposed on grayscale two-dimensional imaging (Figure 34.4A) or three-dimensional imaging (Figure 34.3D).
As with pulsed-wave Doppler, this technique is subject to a Nyquist limit. Velocities are often depicted on a color map as gradations of red and blue, the colors typically used to demonstrate blood movement toward and away from the transducer, respectively.
Tissue Doppler Echocardiography
Tissue Doppler is another pulsed-wave Doppler-based technique, but limits the velocities interrogated to much lower values than with typical pulse or color Doppler. Using this technique allows for velocity of the myocardium to be assessed at specific locations of cardiac structures (more often at the base of the left ventricle and right ventricle).
Strain Echocardiography
Strain imaging monitors tissue deformation over time to assess myocardial function of the different cardiac chambers. In echocardiography, this is typically done using speckle tracking, where bright points within the myocardium are tracked using specialized software to calculate the change in distance between the two points over time.21,22
Strain of the left ventricle can be evaluated in the multiple axes of cardiac motion, including longitudinal (base to apex), circumferential (clockwise or counterclockwise twisting in the short axis), and radial (movement relative to the center of the left ventricle in short axis). Strain imaging can also be performed on other myocardial walls, including the right ventricle, left atrium, and right atrium.
Ultrasound-Enhancing Agents
Echocardiography using ultrasound-enhancing agents is based on the principle that strong ultrasound reflectors can be injected into the blood pool to allow for better visualization of cardiac structures or to more easily evaluate flow patterns.
On the right side of the heart, agitated saline containing small air bubbles is injected through the venous system. When the small air bubbles reach the right side of the heart, a high-intensity signal fills the chambers and appears bright on the echocardiogram. The air bubbles are normally filtered in the pulmonary capillaries and do not appear in the left side of the heart. Presence of bubbles in left-sided chambers raises the possibility of right-to-left shunting, which may be intracardiac or intrapulmonary.
To introduce reflectors into the blood pool on the left side of the heart, microbubbles that are smaller than the pulmonary capillaries must be used to allow entry to the left atrium and ventricle. Several commercial products that consist of small inert gas microbubbles are available. These are imaged using specialized settings (low power) compared to conventional two-dimensional echocardiography because the microbubbles are fragile and are rapidly destroyed by ultrasound waves. Echocardiography with ultrasound-enhancing agents of the left ventricle has allowed for improved visualization of the left ventricular wall motion and EF assessment when imaging windows are difficult, improved detection of apical aneurysms and thrombus, and may be useful in assessing myocardial perfusion.23
CLINICAL APPLICATIONS
Methodology
Ischemic Heart Disease
Ischemic heart disease can be assessed by echocardiography to evaluate abnormalities in wall motion and overall left ventricular EF.24 Cardiac ischemia or infarction may create a regional wall motion, abnormality reflecting the perfusion pattern of the coronary artery. For example, the left anterior descending (LAD) artery typically supplies the anterior wall, apex, and anterior septum with blood. The right coronary artery typically supplies the inferior septum and inferior wall. The left circumflex artery typically supplies the lateral wall. The inferolateral wall can be supplied by either the right coronary or the left circumflex artery.
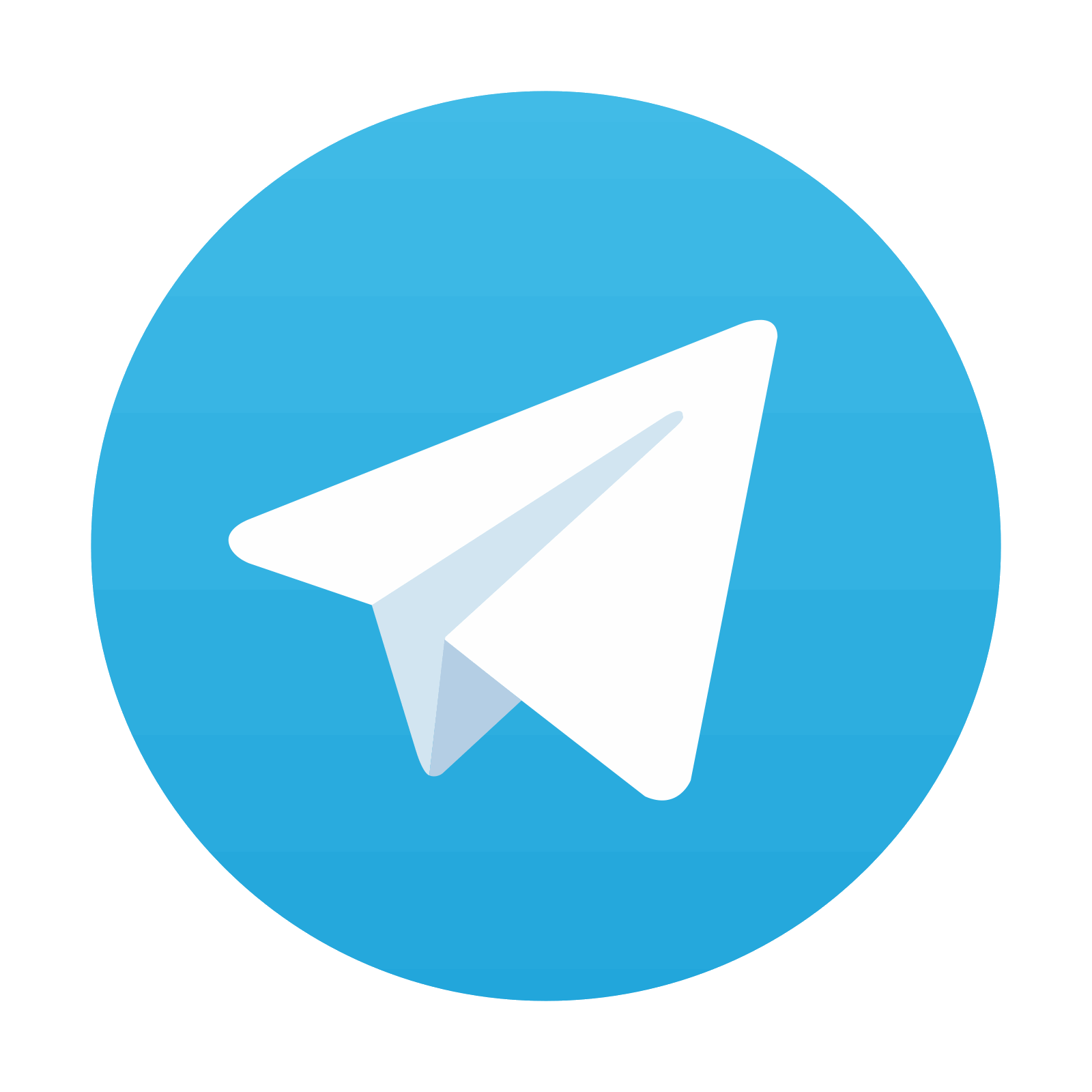
Stay updated, free articles. Join our Telegram channel
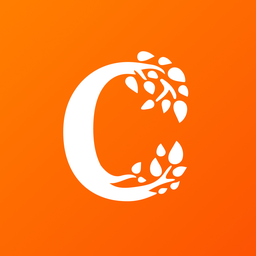
Full access? Get Clinical Tree
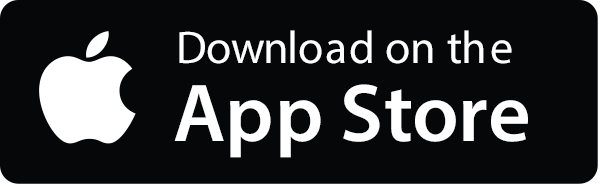
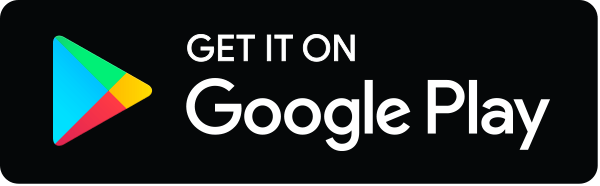
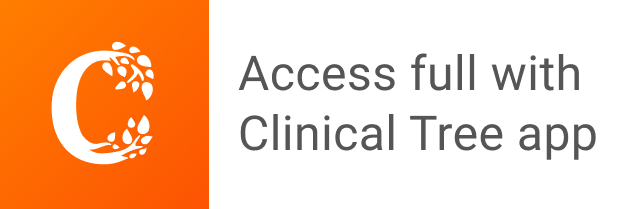