Background
Pronounced echocardiographically measured mechanical dyssynchrony is a positive predictor of response to cardiac resynchronization therapy (CRT), whereas right ventricular (RV) dysfunction is a negative predictor. The aim of this study was to investigate how RV dysfunction influences the association between mechanical dyssynchrony and left ventricular (LV) volumetric remodeling following CRT.
Methods
One hundred twenty-two CRT candidates (mean LV ejection fraction, 19 ± 6%; mean QRS width, 168 ± 21 msec) were prospectively enrolled and underwent echocardiography before and 6 months after CRT. Volumetric remodeling was defined as percentage reduction in LV end-systolic volume. RV dysfunction was defined as RV fractional area change < 35%. Mechanical dyssynchrony was assessed as time to peak strain between the septum and LV lateral wall, interventricular mechanical delay, and septal systolic rebound stretch. Simulations of heart failure with an LV conduction delay in the CircAdapt computer model were used to investigate how LV and RV myocardial contractility influence LV dyssynchrony and acute CRT response.
Results
In the entire patient cohort, higher baseline septal systolic rebound stretch, time to peak strain between the septum and LV lateral wall, and interventricular mechanical delay were all associated with LV volumetric remodeling in univariate analysis ( R = 0.599, R = 0.421, and R = 0.410, respectively, P < .01 for all). The association between septal systolic rebound stretch and LV volumetric remodeling was even stronger in patients without RV dysfunction ( R = 0.648, P < .01). However, none of the mechanical dyssynchrony parameters were associated with LV remodeling in the RV dysfunction subgroup. The computer simulations showed that low RV contractility reduced CRT response but hardly affected mechanical dyssynchrony. In contrast, LV contractility changes had congruent effects on mechanical dyssynchrony and CRT response.
Conclusions
Mechanical dyssynchrony parameters do not reflect the negative impact of reduced RV contractility on CRT response. Echocardiographic prediction of CRT response should therefore include parameters of mechanical dyssynchrony and RV function.
Highlights
- •
The authors investigated the associations among RV function, dyssynchrony, and CRT response.
- •
In patients with RV dysfunction, dyssynchrony was not associated with CRT response.
- •
In computer simulations, dyssynchrony was not affected by RV myocardial dysfunction.
- •
In the simulations, RV myocardial dysfunction limited CRT response.
- •
Measuring RV function can improve prediction of response by mechanical dyssynchrony.
Cardiac resynchronization therapy (CRT) is an established treatment for patients with heart failure and evidence of electrical conduction delay. Despite the success of CRT in large clinical trials, predicting CRT response in individual patients remains challenging. Response prediction is difficult because the mechanisms through which CRT response occurs are still not completely understood. An important mode of action of CRT is the correction of mechanical dyssynchrony caused by an electrical conduction delay, resulting in improvement in myocardial efficiency. Attempts to predict outcome after CRT by identifying such electrical or mechanical substrates have yielded variable results, however.
Right ventricular (RV) function is an important predictor of echocardiographic and clinical outcomes following CRT. The impact of RV function on prognosis has been demonstrated in both observational studies and in landmark CRT trials. RV dysfunction is strongly associated with more advanced heart failure. Moreover, changes in RV function and loading can lead to mechanical dyssynchrony through ventricular interaction, even without underlying electrical dyssynchrony. Whether RV function directly affects mechanical dyssynchrony and CRT response, and how this relates to the association with more advanced heart failure, remains unclear. We therefore used echocardiographic deformation imaging to investigate whether RV dysfunction affects baseline mechanical dyssynchrony indices in a CRT population. We also investigated how these indices related to CRT response (i.e., volumetric remodeling). CRT response was defined as the reduction in left ventricular (LV) end-systolic volume 6 months after CRT. We further hypothesized that RV dysfunction could directly influence both mechanical dyssynchrony and CRT response, independent of LV condition. Because determining causation in the interaction between RV and LV myocardial dysfunction and mechanical dyssynchrony using patient data is challenging, we also performed computer simulations. Simulations were performed with the multiscale CircAdapt model of the human heart and circulation to isolate and explain the effects of RV and LV myocardial dysfunction on both mechanical dyssynchrony and CRT response.
Methods
Study Population and Protocol
The study population consisted of a cohort of prospectively enrolled patients undergoing CRT because of medication-refractory heart failure (New York Heart Association [NYHA] classes II–IV, LV ejection fraction [LVEF] < 35%) and evidence of conduction disturbances (QRS width ≥ 120 msec) with a left bundle branch block (LBBB)–like morphology on surface electrocardiography. Patients were excluded from the analysis if they had poor echocardiographic image quality ( n = 20). Echocardiographic and clinical characteristics were prospectively assessed in all patients before and 6 months after CRT. Care was taken to optimize heart failure medication before the implantation of a CRT device. The execution of the study complied with the principles outlined in the Declaration of Helsinki on research in human subjects and with the procedures of the local medical ethics committee. In compliance with Dutch law, the requirement to obtain written informed consent was waived by the local medical ethics committee, as all echocardiograms and CRT implantations were part of standard clinical care.
Echocardiographic Protocol
All echocardiographic data were obtained using a Vivid 7 ultrasound machine (GE, Chicago, IL). A minimum of three loops were acquired at breath hold and analyzed offline (EchoPAC version 6.0.1; GE). In patients with atrial fibrillation, all parameters are averages over five representative beats.
Two-Dimensional Echocardiography and Doppler Imaging
LVEF, LV end-systolic volume (LVESV), and LV end-diastolic volume (LVEDV) were measured using the biplane Simpson method. Reverse remodeling after CRT was defined as the percentage of reduction in LVESV between echocardiographic examination before and 6 months after CRT implantation. Response was defined as a reduction in LVESV of ≥15%.
Mitral regurgitation effective regurgitant orifice was quantified by the proximal isovelocity surface area method. RV measurements were performed in the apical four-chamber view. RV end-diastolic and end-systolic areas were traced and were used to calculate RV fractional area change (RVFAC). RV dysfunction was defined as RVFAC < 35%. Tricuspid annular plane systolic excursion and transtricuspid pressure gradient were also measured. RVFAC was chosen to define RV dysfunction, as we expected that RVFAC would provide the most adequate estimation of RV function in the presence of mechanical dyssynchrony.
For offline deformation imaging, additional narrow-sector single-wall images of the septum, lateral wall of the left ventricle, and free wall of the right ventricle were prospectively acquired from the standard apical views at 51 to 109 Hz. The onset of the QRS complex was taken as the zero reference for timing and strain measurements. Systole was defined using mitral valve closure and aortic valve closure, derived from Doppler flow patterns. Interventricular mechanical delay was assessed as the delay between pulmonary and aortic valve opening.
Deformation Analysis
Dedicated speckle-tracking software (EchoPAC 2DS version 6.1; GE) was used to derive longitudinal strain curves. The region of interest was placed from base to apex and adapted to match wall thickness. Tracking was visually checked and the region of interest adjusted if necessary. Global longitudinal deformation was calculated over the entire length of the wall. To assess LV dyssynchrony, time to peak strain delay between the septum and the lateral wall (Strain-SL) was calculated. Septal systolic rebound stretch (SRSsept) was determined by summing all systolic stretch following prematurely terminated shortening in the septum, as previously described ( Figure 1 ). Septal strain patterns were also categorized as type I (double-peaked), type II (predominant stretch during ejection), and type III (pseudonormal), as previously described ( Figure 1 ).

Device Implantation
Implantation was performed under local anesthesia. RV and atrial leads were placed transvenously at conventional positions. The LV lead was aimed at a tributary of the coronary sinus overlying the LV free wall. Leads were connected to a CRT defibrillator in all patients.
Computer Simulations
The CircAdapt computational model of the human heart and circulation ( www.circadapt.org ) was used to simulate local ventricular myofiber mechanics and global pump function in the hearts of virtual patients with different degrees of LV and RV contractile weakness and LV conduction delay. More detailed descriptions of both the CircAdapt model and simulations we performed are provided in the Supplemental Methods (available at www.onlinejase.com ).
Simulation of LV and RV Failure at Baseline
The starting point for all simulations of heart failure and CRT was a computer model representing normal healthy adult physiology obtained as described previously. We produced a virtual patient with heart failure and LBBB by imposing a cardiac output of 3.1 L/min, on the basis of the mean value in the patient data ( Table 1 ), with a heart rate of 70 beats/min and mean arterial pressure of 92 mm Hg. We reduced RV and LV myocardial contractility, being the intrinsic ability of myocardial tissue to generate active stress following cross-bridge formation, to 60% of the contractility value in healthy myocardium. LV and RV unloaded wall areas were expanded by 10% to represent eccentric remodeling. An activation delay was imposed within the left ventricle to represent LBBB-like activation ( Supplemental Figure 1 , available at www.onlinejase.com ). The resulting LVEF and RV ejection fraction were 22% and 41%, respectively.
Parameter | RVFAC ≥ 35% ( n = 83) | RVFAC < 35% ( n = 39) | P |
---|---|---|---|
Age (y) | 65 ± 11 | 64 ± 12 | .716 |
Male | 52 (63) | 33 (85) | .019 |
Rhythm | |||
Sinus rhythm | 76 (92) | 30 (77) | .041 |
Atrial fibrillation | 7 (8) | 9 (23) | |
NYHA class | |||
II | 8 (10) | 0 (0) | .005 |
III | 70 (84) | 30 (77) | |
IV | 5 (6) | 9 (23) | |
Medication | |||
β-blocker | 71 (86) | 25 (64) | .010 |
ACE inhibitor or ATII antagonist | 76 (92) | 37 (95) | .717 |
Diuretics | 75 (90) | 38 (97) | .269 |
Aldosterone antagonist | 42 (51) | 23 (59) | .440 |
Lead position | .606 | ||
(Postero)lateral | 68 (82) | 32 (82) | |
Anterolateral | 7 (8) | 5 (13) | |
Posterior | 8 (10) | 2 (5) | |
QRS width (msec) | 170 ± 25 | 167 ± 20 | .517 |
LBBB | 57 (69) | 23 (59) | .313 |
Ischemic etiology | 44 (53) | 11 (28) | .012 |
LVEDV (mL) | 237 ± 65 | 290 ± 107 | .006 |
LVESV (mL) | 188 ± 59 | 247 ± 101 | .001 |
LVEF (%) | 21 ± 6 | 16 ± 5 | <.001 |
Cardiac output (L/min) | 3.1 ± 1.0 | 3.1 ± 1.2 | .902 |
Left atrial size (mm) | 48 ± 8 | 54 ± 7 | <.001 |
MRERO (mm 2 ) | 8 ± 7 | 14 ± 8 | .001 |
SRSsept (%) | 5.2 ± 3.5 | 3.3 ± 2.9 | .003 |
Septal strain pattern | .017 | ||
1 (double-peaked) | 27 (33) | 5 (13) | |
2 (predominant stretch) | 28 (34) | 11 (28) | |
3 (pseudonormal) | 28 (34) | 23 (59) | |
Strain-SL (msec) | 276 ± 143 | 230 ± 145 | .099 |
IVMD (msec) | 47 ± 26 | 47 ± 26 | .983 |
RVEDA (cm 2 ) | 14 ± 5 | 20 ± 5 | <.001 |
RVESA (cm 2 ) | 7 ± 4 | 15 ± 4 | <.001 |
RVFAC (%) | 49 ± 10 | 25 ± 6 | <.001 |
TAPSE (mm) | 19 ± 5 | 14 ± 4 | <.001 |
2DS-RV (%) | −20.4 ± 5.6 | −14.3 ± 4.4 | <.001 |
TRPG (mm Hg) | 30 ± 8 | 35 ± 9 | .009 |
Beginning with the initial virtual patient with heart failure and LBBB, further worsening of RV or LV myocardial contractile weakness was simulated through further reductions in either the contractility of the RV or LV free wall from 60% down to 20% of the original healthy contractility in nine steps. This protocol resulted in nine virtual patients with varying degrees of LV myocardial dysfunction causing a decrease in LVEF to a minimum of 10% and nine virtual patients with varying degrees of RV myocardial dysfunction causing a gradual decrease in RV ejection fraction to a minimum of 24%. Cardiac output, heart rate, and mean arterial pressure were sustained through homeostatic control mechanisms in each virtual patient. The average myofiber strain from the septal wall segments was used to calculate SRSsept for each simulation.
Simulation of CRT
CRT was simulated in each virtual patient using an activation pattern representing preexcitation of the RV apex and the LV lateral wall, as shown in Supplemental Figure 1 (available at www.onlinejase.com ). When simulating the acute effects of CRT, homeostatic control was turned off to allow changes in stroke volume to occur. We defined the acute response to CRT in the simulations as the percentage change in stroke volume after initiation of CRT once a new hemodynamic steady state was reached.
Statistical Analysis
Statistical analysis was performed using SPSS (SPSS, Chicago, IL). Values are presented as mean ± SD for continuous variables and as numbers and percentages for categorical variables. Continuous data were compared using the paired or unpaired t test as appropriate. Categorical data were compared using the χ 2 or Fisher exact test. Correlations between parameters were expressed using Pearson or Spearman correlation coefficients as appropriate. Univariate analysis of parameters of LV function, RV function, and dyssynchrony was performed to determine their relation to CRT response. The single best performing parameter for each aspect (i.e., LV function, RV function, and dyssynchrony) was used in a stepwise, forward selection, multivariate model. Three models were used to test whether baseline RV function affected the prediction of reverse remodeling independent of baseline LV function (i.e., LVEDV, LVESV, or LVEF) and mechanical dyssynchrony. One model incorporated the best performing dyssynchrony and LV function parameter, one incorporated the best performing dyssynchrony and RV function parameter, and one incorporated all three. A P value < .05 was considered to indicate statistical significance for all analyses.
Measurement Variability
Last, measurement variability of key echocardiographic parameters and dyssynchrony parameters was analyzed by a second observer in 20 randomly selected patients and compared using the intraclass correlation coefficient. Intraclass correlation coefficient values were as follows, 0.98 for LVEDV, 0.97 for LVESV, 0.77 for LVEF, 0.83 for RV end-diastolic area, 0.90 for RV end-systolic area, 0.93 for RVFAC, 0.91 for interventricular mechanical delay, 0.75 for Strain-SL, and 0.92 for SRSsept.
Results
Patient Study
Study Population
Table 1 shows baseline characteristics of patients with and without RV dysfunction. Patients with RV dysfunction had more advanced heart failure with more pronounced RV and LV dilatation, lower LVEFs, larger left atria, more severe mitral regurgitation, and higher NYHA functional class. These patients were also more often male, more often had atrial fibrillation, had higher transtricuspid pressure gradients, and less often had an ischemic etiology of heart failure. Of all dyssynchrony parameters, only SRSsept was significantly lower in patients with RV dysfunction.
CRT Response
In total, nine patients died (four patients without RV dysfunction and five with RV dysfunction), and three underwent LV assist device implantation or heart transplantation (two patients without RV dysfunction and one with RV dysfunction) before the 6-month follow-up visit. NYHA class decreased by two levels in nine patients (7%), decreased by one level in 65 patients (53%), remained stable in 33 patients (27%), and had missing data in 15 patients (12%). NYHA class decreased significantly compared with baseline values in the subgroup without RV dysfunction. However, there was no statistically significant difference in change of NYHA class between the two subgroups ( Table 2 ). LV volumes could not be quantified at 6-month follow-up in three patients. In the remaining population, CRT reduced LVEDV (from 256 ± 84 to 227 ± 96 mL) and LVESV (from 208 ± 80 to 174 ± 92 mL) and improved LVEF (from 20 ± 7% to 26 ± 11%) at 6 months ( P < .001 for all). RV free wall peak strain (−18.2% to −21.1%, P < .001), RV end-systolic area (10 ± 6 to 9 ± 4 cm 2 , P < .05), and transtricuspid pressure gradient (33 ± 9 to 29 ± 9 mm Hg, P < .05) showed a significant improvement after CRT, whereas RVFAC and tricuspid annular plane systolic excursion did not. Table 2 shows responses in patients with and without RV dysfunction. Patients with RV dysfunction showed less LV reverse remodeling and less improvement of LVEF, but improvements in RV parameters were greater in these patients. There were nine volumetric responders (28%) in the subgroup with RV dysfunction, compared with 45 (60%) in the subgroup without RV dysfunction ( P < .01).
Parameter | RVFAC ≥ 35% ( n = 83) | RVFAC < 35% ( n = 39) | P |
---|---|---|---|
ΔLVEDV (%) | −17 ± 19 ∗ | −3 ± 16 | <.001 |
ΔLVESV (%) | −24 ± 23 ∗ | −7 ± 18 ∗ | <.001 |
ΔLVEF (percentage points) | 8 ± 8 ∗ | 4 ± 7 ∗ | .006 |
Δ2DS-RV (percentage points) | −2.3 ± 6.0 ∗ | −4.4 ± 4.7 ∗ | .082 |
ΔRVEDA (%) | 8 ± 31 | −15 ± 34 ∗ | .001 |
ΔRVESA (%) | 18 ± 44 | −25 ± 30 ∗ | <.001 |
ΔRVFAC (percentage points) | −4 ± 11 ∗ | 9 ± 11 ∗ | <.001 |
ΔTRPG (mm Hg) | −2 ± 9 | −5 ± 7 ∗ | .343 |
ΔTAPSE (mm) | −1 ± 4 ∗ | 2 ± 4 ∗ | <.001 |
ΔNYHA class | |||
0 | 24 (32) ∗ | 9 (28) | .913 |
−1 | 45 (60) ∗ | 20 (63) | |
−2 | 6 (8) ∗ | 3 (9) |
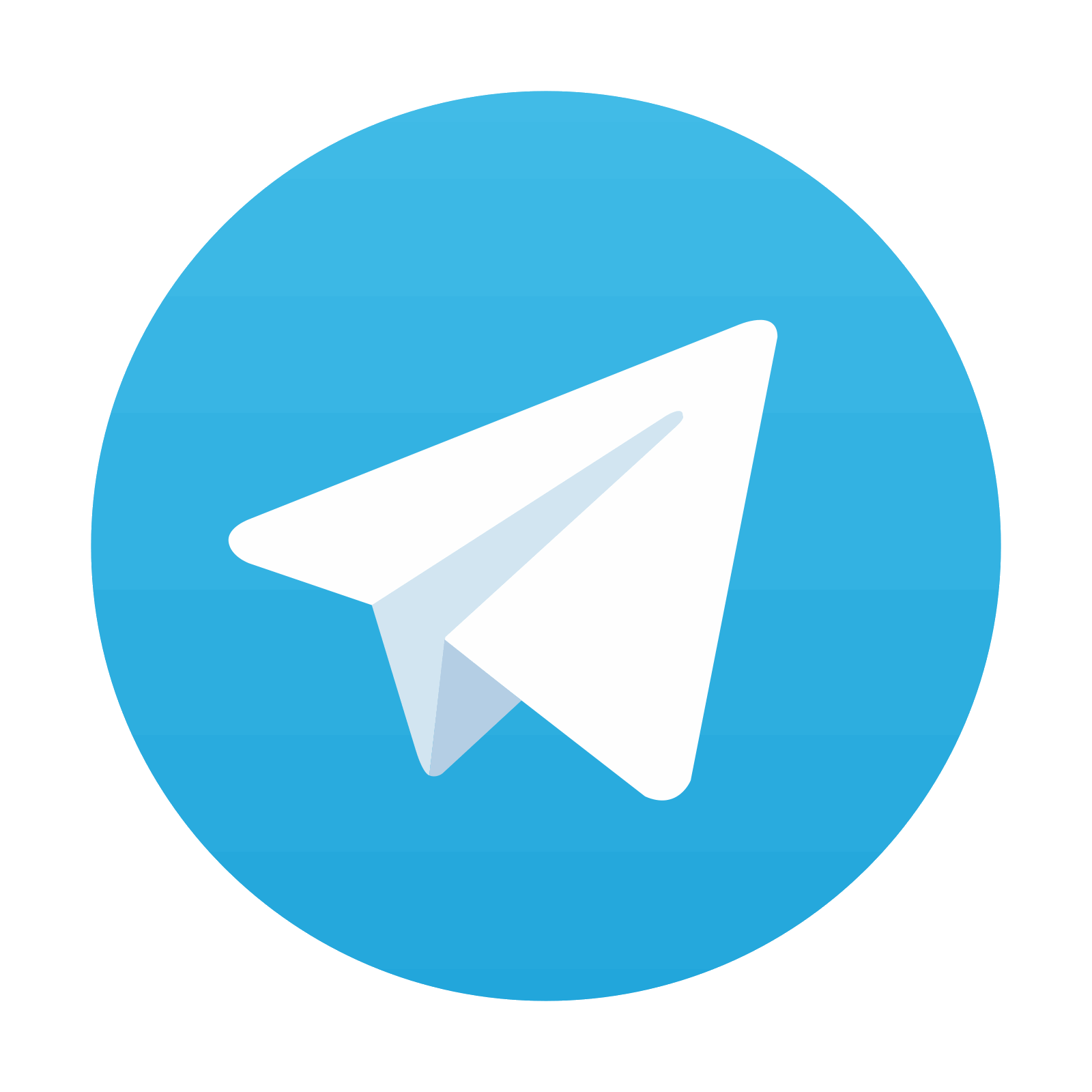
Stay updated, free articles. Join our Telegram channel
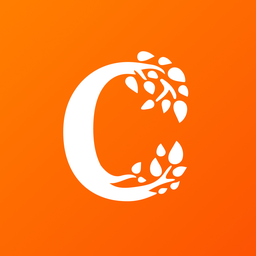
Full access? Get Clinical Tree
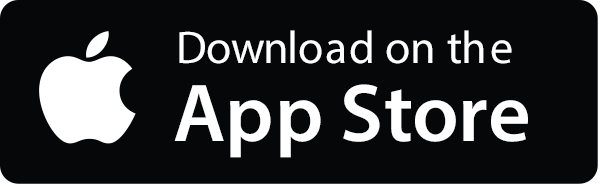
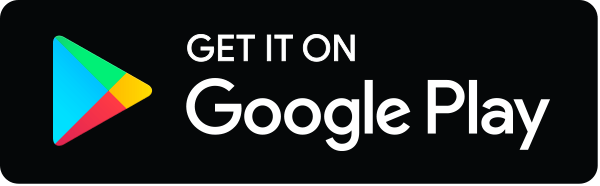
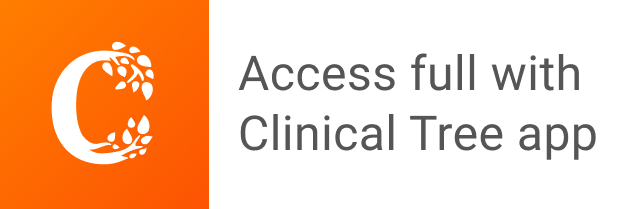