Fig. 5.1
Normal size of thoracic aortic segments. The thoracic aorta can be divided into three segments: the ascending aorta that extends from the aortic annulus to the innominate artery and is typically measured at the level of the aortic annulus, the sinuses of Valsalva, the sinotubular junction, and the proximal ascending aorta; the aortic arch that extends from the innominate artery to the ligamentum arteriosum; and the descending aorta that extends from the ligamentum arteriosum to the level of the diaphragm. PA right pulmonary artery
5.1.1.4 Aortic Pressure-Dimension Relationship
An increase in distending pressure during systole induces an increase in aortic dimension which is directly related to the elastic properties of the aorta [7]. Diameter or area changes can be determined using TTE or TOE, but the estimation of the pressure changes at the same site may be unreliable because of the amplification of the pulse pressure and the inaccuracy of all cuff sphygmomanometer systems, particularly in young subjects. However, studies have shown a good correlation between aortic distensibility calculated using echocardiography and noninvasive brachial artery blood pressure (BP) measurements and aortic distensibility calculated invasively using contrast aortography and direct aortic pressure recordings. Changes in arterial diameter can be measured at the level of the ascending aorta ~3 cm above the aortic valve in 2D-guided M-mode of parasternal long-axis view, with the diastolic aortic diameter measured at the peak of the QRS complex and systolic aorta diameter measured at the maximal anterior motion of the aorta [8, 9].
There are several indices derived from aortic or arterial dimensions and pressures, used for the estimation of the elastic properties of the aorta. The indices most frequently used are the aortic/arterial distensibility (the relative change in a diameter or area for pressure change), the compliance (the absolute change in diameter or area for pressure change), and the non-dimensional index of local arterial stiffness named the β-index which is less affected by arterial pressure changes and is easily measured using echo-tracking devices [10].
5.1.2 The Aorta and Blood Pressure
Although early case reports and pathological series suggest that hypertension might directly contribute to the enlargement of the aorta, more recent pathological and M-mode echocardiographic studies have not found an association between BP and aortic size when the confounding influence of aging is considered. Thus, aortic diameter is strongly related to age, and senescence may result in cystic medial necrosis [11]. In contrast, other M-mode echocardiographic studies have noted significant relations of aortic diameter to systolic and diastolic BP.
As mentioned before, studies evaluating the relation of aortic root size to BP have yielded conflicting results [12]. In the Framingham Heart Study, scientists using two-dimensionally guided M-mode measurement of the sinuses of Valsalva found that diastolic pressure was directly related to aortic diameter, whereas both systolic and pulse pressures were inversely related to aortic diameter after adjustment for age, height, and weight [13]. In the Cardiovascular Health Study, a relation was found between diastolic, but not systolic, BP and M-mode echocardiographic aortic dimensions when the entire elderly cohort was analyzed. However, when the healthier subgroup was examined (subjects not receiving antihypertensive therapy or without coronary heart disease), aortic diameter was not associated with BP. Other researchers have shown either no relation of BP to M-mode aortic measurements or a relationship only to systolic BP [14].
There are few studies showing a correlation between aortic root diameter and hemodynamic load. A progressive enlargement in aortic dimension was found only at the supra-aortic ridge and ascending aorta according to the quartiles of casual systolic blood pressure, while all aortic diameters were found to significantly increase according to the quartiles of casual diastolic pressure, with the most significant increases occurring in the more distal segments [15].
Four previous studies have reported inverse associations between aortic diameter and pulse pressure (PP). In the Framingham cohort an independent inverse association was found between aortic diameter and PP, and it was speculated that intimal-medial hypertrophy or reflex smooth muscle activation induced by an increased PP may underlie this association [13]. Similarly, a substudy of the Losartan Intervention for Endpoint Reduction Trial observed an inverse association between aortic diameter and PP and hypothesized that the higher compliance of the aortic root may reduce the rise in systolic pressure and thus result in lower PP [16]. In a subgroup of the stroke prevention, an inverse association between aortic diameter and PP was also found. Furthermore in a smaller study, it was shown that aortic dimensions were inversely associated with carotid PP (but not brachial) in the healthy control group but were positively associated with PP in subjects with Marfan syndrome who have known alterations in their aortic wall properties. The authors speculated that this inverse association between aortic diameter and carotid PP in the healthy cohort was due to the reduced wave reflection, accruing from the higher aortic compliance of subjects with larger aortic diameters [17, 18].
5.1.3 Relationship Between 24-h Ambulatory Blood Pressure and Aortic Dimensions
The impact of ambulatory BP and its circadian components on aortic diameter in hypertensive patients has never been prospectively investigated in large studies. In a small series of 48 hypertensive patients, it was shown that awake diastolic BP was independently correlated with aortic diameter measured at four different locations [19]. In a study of a larger sample, it was observed that nocturnal BP may have an independent role in determining aortic dimension. This observation is further supported by the progressive reduction in the prevalence of a normal nocturnal BP pattern with advancing aortic root size. This finding is also in line with the evidence that nocturnal BP has superior prognostic value than awake BP in predicting cardiovascular morbidity and mortality in both normotensive and hypertensive individuals. The reasons of the prognostic superiority of nighttime over daytime BP are not clear. However, there is a hypothesis suggesting that the excessive variability of diurnal BP may reduce its prognostic value compared to that of nocturnal BP [20].
Individuals with obstructive sleep apnea (OSA) are usually non-dippers and have aortic dilatation in echocardiography. During each apneic episode there is a marked increase in transmural pressure of the aortic wall, resulting in aortic dilatation. An animal study showed that the thoracic aorta can be distended during the diastolic phase in the setting of negative intrathoracic pressure, probably due to diminished antegrade flow out of the thorax. Furthermore, cyclical fluctuations in sympathetic activity and BP, which have been shown to progressively increase during apneic episodes in patients with OSA, may result in aortic dilatation [21, 22].
5.1.4 Modifications of the Aorta and Cardiovascular Events
Although anatomical changes of the aortic root are likely to reflect the effects of hypertension and atherosclerosis, few data are available on the predictive value of aortic root dimension for cardiovascular events. In the Cardiovascular Health Study, it was shown that a large aortic root dimension is associated with an increased risk for incident congestive heart failure, stroke, and cardiovascular mortality but not for myocardial infarction. The lack of association between aortic root dimension and incident myocardial infarction may suggest that an increased aortic root is not a consequence of acute myocardial infarction, but of the interaction of age, hypertension, and atherosclerosis over time [23]. The Rotterdam Study observed that the presence of atheromatous plaques in the aorta was strongly correlated with decreased aortic distensibility. In contrast, other studies have found no relation between arterial stiffness and aortic atherosclerosis. However, the elevation of systolic blood pressure, causing a rise in left ventricular afterload and myocardial work, and the decrease in diastolic blood pressure, which reduces coronary perfusion, result in subendocardial ischemia [24, 25].
5.2 Coronary Arteries
5.2.1 Coronary Artery Remodeling in Hypertension
In essential hypertension remodeling of the small arteries (100–300 mm of diameter) is the most prevalent and earliest form of target organ damage. At the level of the coronary artery tree, it causes increased vascular reactivity and a reduction in coronary flow reserve (CFR) at maximal vasodilation [26–28]. Coronary remodeling in the form of media thickening is mainly caused by complex hemodynamic and neurohumoral interactions [26, 28]. The dimensions of the epicardial coronary arteries remain constant resulting in an elevated coronary flow velocity [29]. This may increase longitudinal shear stress, causing premature atherosclerosis. It should be noted that changes in microcirculation maintain the increase in vascular resistance that is common in hypertension [30]. However, it is still under investigation whether these microcirculatory abnormalities are the cause or effect in the hypertensive setting [31].
Blood vessels react to physiologic or pathologic conditions with changes in their size and structure [32]. Exposure of the large coronary arteries to high pulsatile pressure and blood flow velocity can lead to an increase in endothelial shear stress, resulting in endothelial dysfunction responsible for the increase in the endothelial permeability and deposition of lipoproteins in the arterial wall and eventually the formation of atherosclerotic plaques [33].
Coronary remodeling in hypertensive patients can be distinguished as positive and negative. Compensatory or positive remodeling (described by Glagov) delays the onset of luminal narrowing because the vessel expands with plaque enlargement [34], while in the case of negative remodeling, the vessel’s cross-sectional area is much less compared to the adjacent normal reference segment contributing to the development of focal coronary artery stenosis [35]. Based on the presence or not of stenosis, coronary remodeling may be also defined as adequate or inadequate, respectively.
Arterial remodeling is considered to be initiated by the detection of signals related to changes in hemodynamic conditions such as blood flow, wall stretch, and shear stress as well as signals related to humoral factors that include cytokines and vasoactive substances [32]. These signals which are relayed to the endothelium and transmitted to adjacent cells can lead to the synthesis or the activation of substances that influence cell growth and apoptosis. The extracellular matrix also appears to have an important role in the remodeling response on account of a group of enzymes (matrix metalloproteinases) that regulate the composition of the matrix by selective degradation of its components. Inflammation plays a key role in coronary remodeling and the atherosclerotic process, contributing to the formation of the vulnerable plaque prone to rupture leading to acute coronary syndromes [32, 34]. In contrast, fibrotic changes could lead to a reduced risk of plaque rupture and to plaque stabilization.
5.2.2 Methods for Assessment
Although contrast coronary angiography offers excellent visualization of the configuration of the long axes of the blood vessel lumen, it lags in information on the structure, morphology, and consistence of the coronary arterial wall [35, 36]. Intravascular ultrasound (IVUS) can provide information on the arterial wall under the endothelial surface and can help detect structural and functional changes of the coronary vessels in hypertensive patients [37]. By using IVUS in the coronary arteries, we can evaluate the lumen area and geometry, the wall tissue characteristics and thickness, the branch points, the degree of calcification, and the location and the extent of atherosclerotic lesions [38, 39]. Occult plaques can be concealed in segments of an apparent and angiographically normal coronary artery, and only intravascular imaging modalities like IVUS can identify them [34].
Intravascular US consists of an ultrasound catheter that incorporates a polyethylene shaft and a phased array transducer tip. The multielement array yields an image perpendicular to the axis of the catheter. The field of view is adjustable from 8 to 16 mm in diameter, and images may be acquired up to 10 frames/second [38].
Intravascular US studies have demonstrated that arterial remodeling could be bidirectional and it is a plaque-specific rather than a patient-specific process. That means that in the same coronary artery, the degree of the remodeling varies from plaque to plaque [34]. The use of IVUS is valuable to understand the nature and the characteristics of the coronary wall during the process of remodeling. Indeed IVUS imaging has demonstrated an expansion or a shrinkage of the external elastic membrane area in the case of positive or negative remodeling, respectively [32].
5.2.3 Coronary Circulation: Functional Changes
In hypertensive patients, the structural alterations of the intramyocardial arteries of the coronary tree contribute to the reduced coronary vasodilator capacity and to an increased minimal coronary resistance independently of the presence of left ventricular hypertrophy [37]. Studies have documented a reduced coronary reserve in most of the hypertensive patients even in the absence of coronary artery disease. Responsible of this impaired coronary vasodilator reserve are considered mainly the intramyocardial arterioles that largely contribute in the coronary vascular resistance and the autoregulation of myocardial perfusion. Arterial hypertension increases the wall-to-lumen ratio of the intramyocardial arteries by inducing arterial medial hypertrophy that in turn leads to wall thickening and lumen reduction. Moreover, the coronary vasodilator capacity can be influenced by vascular tone, endothelial dysfunction, increase of vascular collagen biosynthesis, interstitial and periarteriolar fibrosis, or/and noxious interactions between structural and functional components of a thickened arterial wall. In addition, studies have revealed that the lumen size of the major coronary arteries of hypertensive patients do not match with the increased coronary flow [40]. This mismatch can be influenced by the vascular tone, but it is more related to the absence of structural enlargement.
5.2.4 How to Perform Coronary Flow Reserve Measurements
It is established that microvascular function can be accurately assessed by CFR measured either invasively [41] or noninvasively [42].
5.2.4.1 Invasive
A 0.014 in. Doppler guidewire (FloWire, Cardiometrics) is advanced into the proximal part of the left anterior descending artery. ECG, coronary ostial pressure, instantaneous spectral peak velocity, and time-averaged spectral peak flow velocity are continuously and simultaneously recorded. Then an intracoronary bolus of 60 μg adenosine is administered into the left coronary artery, and further measurements are obtained under peak hyperemic conditions. CFR is calculated as the ratio of hyperemic to baseline time-averaged spectral peak flow velocity. All measurements should be performed twice, and mean values should be calculated.
5.2.4.2 Noninvasive
Parts of coronary arteries can be visualized using TTE. The anatomy and pathophysiology of the coronary arteries can be rivaled based on two-dimensional evaluation, but the introduction of Doppler velocity measurements has allowed the assessment of the function of the coronary arteries, and TTE has been introduced in the detection of coronary flow velocity and coronary flow velocity reserve (CFVR) [42]. CFR can be quantified using flow velocity-derived methods. Three types of Doppler techniques can be applied to coronary flow measurement: pulse wave Doppler in which intermittent bursts of ultrasound are transmitted, continuous wave Doppler where ultrasounds are transmitted and received continuously, and color flow mapping which uses simple sampling volumes to record shifts [42].
CFR can be quantificated by inducing maximal hyperemia with the use of pharmacological agents such as adenosine, ATP, and dipyridamole. CFR can be affected by epicardial stenosis and microcirculation. In the absence of stenosis in the epicardial arteries, CFVR depicts the reactivity of the microcirculation. To level out the effect of microcirculation on CFR measurements in stenosed vessels, the relative flow reserve has been developed. It is calculated by comparing the flow velocity reserve distal to the stenosis in the target vessel with the velocity reserve to a nonstenosed reference vessel [42].
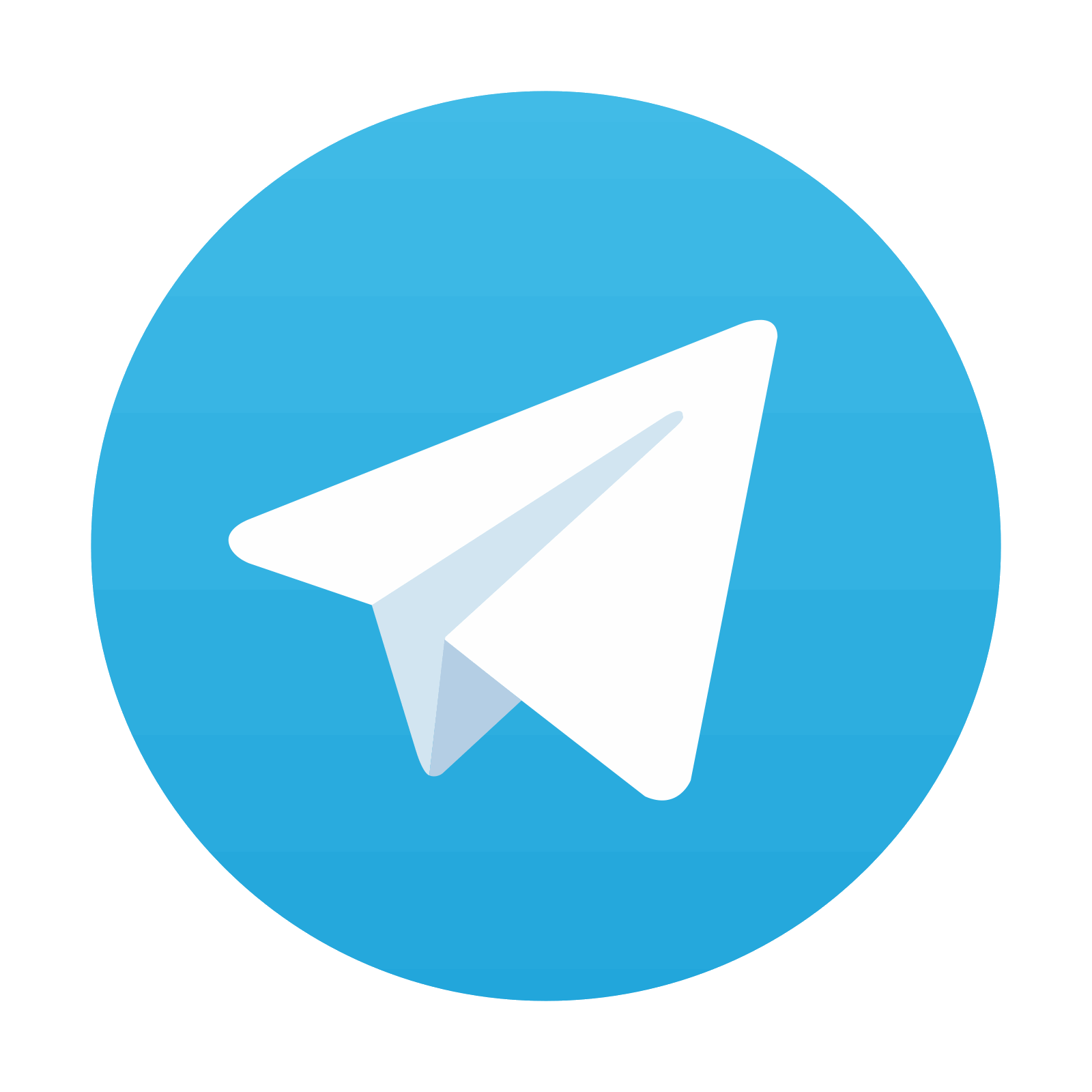
Stay updated, free articles. Join our Telegram channel
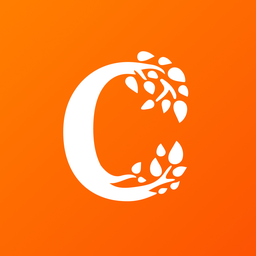
Full access? Get Clinical Tree
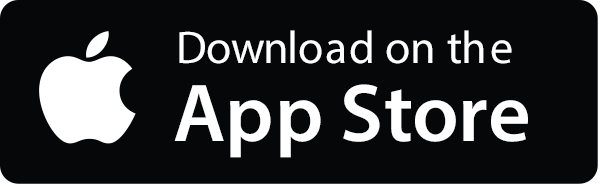
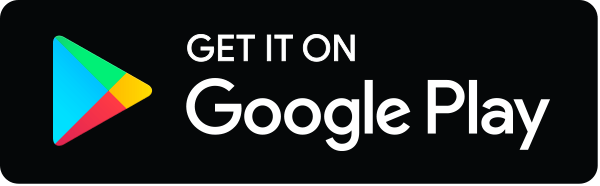