Attention ASE: Members:
ASE has gone green! Visit www.aseuniversity.org to earn free continuing medical education credit through an online activity related to this article. Certificates are available for immediate access upon successful completion of the activity. Nonmembers will need to join ASE to access this great member benefit!
1
Introduction
Three-dimensional (3D) echocardiographic (3DE) imaging represents a major innovation in cardiovascular ultrasound. Advancements in computer and transducer technologies permit real-time 3DE acquisition and presentation of cardiac structures from any spatial point of view. The usefulness of 3D echocardiography has been demonstrated in (1) the evaluation of cardiac chamber volumes and mass, which avoids geometric assumptions; (2) the assessment of regional left ventricular (LV) wall motion and quantification of systolic dyssynchrony; (3) presentation of realistic views of heart valves; (4) volumetric evaluation of regurgitant lesions and shunts with 3DE color Doppler imaging; and (5) 3DE stress imaging. However, for 3D echocardiography to be implemented in routine clinical practice, a full understanding of its technical principles and a systematic approach to image acquisition and analysis are required. The main goal of this document is to provide a practical guide on how to acquire, analyze, and display the various cardiac structures using 3D echocardiography, as well as limitations of the technique. In addition, this document describes the current and potential clinical applications of 3D echocardiography along with their strengths and weaknesses.
1
Introduction
Three-dimensional (3D) echocardiographic (3DE) imaging represents a major innovation in cardiovascular ultrasound. Advancements in computer and transducer technologies permit real-time 3DE acquisition and presentation of cardiac structures from any spatial point of view. The usefulness of 3D echocardiography has been demonstrated in (1) the evaluation of cardiac chamber volumes and mass, which avoids geometric assumptions; (2) the assessment of regional left ventricular (LV) wall motion and quantification of systolic dyssynchrony; (3) presentation of realistic views of heart valves; (4) volumetric evaluation of regurgitant lesions and shunts with 3DE color Doppler imaging; and (5) 3DE stress imaging. However, for 3D echocardiography to be implemented in routine clinical practice, a full understanding of its technical principles and a systematic approach to image acquisition and analysis are required. The main goal of this document is to provide a practical guide on how to acquire, analyze, and display the various cardiac structures using 3D echocardiography, as well as limitations of the technique. In addition, this document describes the current and potential clinical applications of 3D echocardiography along with their strengths and weaknesses.
2
Instrumentation
a
Fully Sampled Matrix-Array Transducers
An important milestone in the history of real-time 3D echocardiography was reached shortly after the year 2000, with the development of fully sampled matrix-array transducers. These transducers provided excellent real-time imaging of the beating heart in three dimensions and required significant technological developments in both hardware and software, including transducer design, microelectronic techniques, and computing.
Currently, 3DE matrix-array transducers are composed of nearly 3,000 piezoelectric elements with operating frequencies ranging from 2 to 4 MHz and from 5 to 7 MHz for transthoracic echocardiographic (TTE) and transesophageal echocardiographic (TEE) imaging, respectively. These piezoelectric elements are arranged in a matrix configuration within the transducer and require a large number of digital channels for these fully sampled elements to be connected. To reduce both power consumption and the size of the connecting cable, several miniaturized circuit boards are incorporated into the transducer, allowing partial beam-forming to be performed in the probe. Additionally, developments in transducer technology have resulted in a reduced transthoracic transducer footprint, improved side-lobe suppression, increased sensitivity and penetration, and the implementation of harmonic capabilities that can be used for both grayscale and contrast imaging. The most recent generation of matrix transducers are significantly smaller than the previous ones, and the quality of two-dimensional (2D) and 3D imaging has improved significantly, allowing a single transducer to acquire both 2D and 3DE studies.
3
Data Acquisition
Currently, there are two different methods for 3DE data acquisition: real-time or live 3DE imaging and electrocardiographically triggered multiple-beat 3DE imaging. Real-time or live 3DE refers to the acquisition of multiple pyramidal data sets per second in a single heartbeat. Most ultrasound systems have real-time 3DE volume imaging available in the following modes: live 3D narrow volume, live 3D zoomed, live 3D wide angled (full volume), and live 3D color Doppler. Although this methodology overcomes the limitations imposed by rhythm disturbances or respiratory motion ( Figure 1 ), it is limited by poor temporal and spatial resolution.
In contrast, multiple-beat 3D echocardiography provides images of higher temporal resolution. This is achieved through multiple acquisitions of narrow volumes of data over several heartbeats (ranging from two to seven cardiac cycles) that are subsequently stitched together to create a single volumetric data set ( Figure 2 ). However, gated imaging of the heart is inherently prone to imaging artifacts created by patient or respiratory motion or irregular cardiac rhythms.
Data Acquisition Modes
Simultaneous Multiplane Mode
Simultaneous multi-plane imaging is unique to the matrix array transducer and permits the use of a dual screen to simultaneously display two real-time images. The first image is typically a reference view of a particular structure, while the second image or “lateral plane” represents a plane rotated 30 to 1500 from the reference plane. Multiplane imaging in the elevation plane is also available. Color flow Doppler imaging can also be superimposed onto the 2D images.
Real-Time 3D Mode—Narrow Sector
Live 3D using the matrix array transducer permits a real-time display of a 300 x 600 pyramidal volume. While the size of the sector is usually insufficient to visualize the entirety of a single structure in any one imaging plane, the superior spatial and temporal resolution permits accurate diagnoses of complex pathologies while preserving optimal temporal resolution.
Focused Wide Sector—“ZOOM”
The “ZOOM” mode permits a focused, wide sector view of cardiac structures. It must be noted that enlarging the region of interest excessively will result in a further detrimental decrease of the spatial and temporal resolution relative to real-time 3DE.
Full Volume—Gated Acquisition
The full volume mode has the largest acquisition sector possible, which is ideal when imaging specific structures such as the mitral valve or aortic root. This mode also has optimal spatial resolution, which permits detailed diagnosis of complex pathologies. As well, it has high temporal resolution (>30 Hz). Similar to the real-time 3D and the focused wide sector—“ZOOM” modalities, the gated full volume can also be rotated to orient structures such as valves in unique en face views. Furthermore, the full volume data set can be cropped or multiplane transected to remove tissue planes in order to identify components of valvular structures within the volume or to visualize 2D cross-sectional x, y, and orthogonal planes using off-line analysis software.
Full Volume with Color Flow Doppler
When 3DE color flow Doppler imaging was first introduced using a matrix array transducer, it could only be displayed using a full volume, gated reconstruction technique. This required the incorporation/“stitching“ of 7-14 individual pyramidal volume slabs gated to the ECG, to create a 3D composite volume, in the upper-end range of a 400 x 400 sector at a frame rate of 15-25 Hz depending upon the selected line density. However, currently 3D color full volume can be acquired with less than the 7-14 individual gated volumes and the most recently developed software allows acquisition of as low as 2 beats, albeit at the cost of temporal resolution.
a
Challenges with 3DE Acquisition
Temporal Versus Spatial Resolution
The main trade-off in 3DE imaging is between volume rate (i.e., temporal resolution) and spatial resolution. To improve spatial resolution, an increased number of scan lines per volume (scan line density) is required, which takes longer to acquire and process and thereby limits the overall volume rate. Fortunately, imaging volumes can be adjusted in size (i.e., made smaller) to increase volume rate while maintaining spatial resolution. Because of the frequent artifacts associated with gating, ultrasound companies are developing real-time technology associated with methods for improving the ultrasound system processing power needed to provide full volume (90° × 90°) real-time 3DE data sets with adequate spatial and temporal resolution.
ECG Gating and Breath Hold
Gated data sets are most challenging in patients with arrhythmias and/or respiratory difficulties. Figure 3 is an example of a 2D depiction of an artifact caused by gated 3DE acquisition. Note that the data set shown in the left panel of Figure 3 appears to be free of artifacts, whereas the image in the right panel has distinct stitching artifacts. If the gated acquisition acquires sector slices in a sweeping motion parallel to the reference image, then every image parallel to the reference image will appear normal. Gating artifacts are most prominent when the volumetric data set is viewed from a cut plane perpendicular to the sweep plane. Methods to minimize the effects of gating artifacts are described in Table 1 . As well, the ECG tracing needs to be optimized to obtain a distinct R wave. Because the most frequent artifacts of gated acquisitions are stitching artifacts, the number of acquisition beats should be tailored to the clinical question to be addressed, taking into account that with more beats, the volume will be wider and the temporal resolution higher. To improve spatial resolution (i.e., the number of scan lines per volume), the pyramidal volume should be optimized to acquire the smaller volume able to encompass the cardiac structure of interest. Before 3DE acquisition, the 2D image should be optimized: “ suboptimal 2D images result in suboptimal 3DE data sets .”
3D Optimization
Low gain settings result in echo dropout, with the potential of artificially eliminating anatomic structures that cannot be recovered during postprocessing. Alternatively, with excess gain, there is a decrease in resolution and a loss of the 3D perspective or depth within the data set. As a general rule, both gain and compression settings should be set in the midrange (50 units) and optimized with slightly higher time gain controls (time gain compensation) to enable the greatest flexibility with postprocessing gain and compression. Table 1 illustrates the issue of overgaining as well as undergaining. Therefore, it is recommended to slightly overcompensate the brightness of the image with time gain compensation rather than using the power-output gain. Using the postprocessing controls allows adjustments between high and low gain settings. However, it is important to note the even distribution of gain using the time gain compensation controls, as uneven areas of brightness cannot be compensated or corrected using postprocessing controls. As with 2D echocardiography, optimizing lateral and axial resolution remains equally important during 3DE acquisition.
4
3DE Image Display
a
Cropping
The concept of cropping is inherent to 3D echocardiography. In contrast to cross-sectional (i.e., tomographic) modalities, 3D echocardiography requires that the “viewing perspective” be in the chamber that is in immediate continuity with the region of interest. For example, to view the atrioventricular junctions “en face,” the operator must crop off the base and the apex of the heart, so that the operator may visualize the junctions looking up from below, or looking down from above. Similarly, to view the ventricular septum en face, the echocardiographer must crop off the free walls of both ventricles to view the right ventricular (RV) aspect of the septum from right to left or the LV aspect of the septum from left to right. The paradigm for the echocardiographer, therefore, is to change from the cross-sectional approach to that of the anatomist or surgeon, who can only view intracardiac structures after exposing them, by cropping the walls of the different chambers. Three-dimensional cropping can be performed either before (during) or after data acquisition. Cropping that is performed before the acquisition has the advantages of providing better temporal and spatial resolution, while also providing immediate availability of the cropped image. However, if a cropped image is stored, that image may not be amenable to “uncropping” later. In contrast, if a wide data set is acquired and cropped after acquisition, it provides the advantage of retaining more diagnostic information, but at the expense of loss of spatial and temporal resolution.
b
Post-Acquisition Display
Once a 3DE data set is acquired, it can be viewed interactively using a number of 3D visualization and rendering software packages. Display of 3DE images can be divided into three broad categories: (1) volume rendering ( Figure 4 A), (2) surface rendering (including wireframe display; Figures 4 B and C), and (3) 2D tomographic slices ( Figure 4 D). The choice of the display technique is generally determined by the clinical application.
c
Volume Rendering
Volume rendering is a technique that uses different types of algorithms (e.g., ray casting, shear warp, and others) to preserve all 3DE information and project it, after processing, onto a 2D plane for viewing. Essentially, these algorithms cast a light beam through the collected voxels. Then, all voxels along each light beam are weighted to obtain a voxel gradient intensity that integrated with different levels of opacification, shading and lighting allows an individual structure to appear solid (i.e., tissue) or transparent (i.e., blood pool). Finally, a variety of shading techniques (distance shading, gray-level gradient coding, and texture shading) are used to generate a 3D display of the depths and textures of cardiac structures.
Volume-rendered 3DE data sets can be electronically segmented and sectioned. To obtain ideal cut planes, the 3D data set can be manipulated, cropped, and rotated. Volume rendering provides complex spatial relationships in a 3D display that is particularly useful for evaluating valves and adjacent anatomic structures.
d
Surface Rendering
Surface rendering is a visualization technique that shows the surfaces of structures or organs in a solid appearance. To use this technique, segmentation of the data set can be applied to identify the structure of interest. Surface rendering of selected structures is obtained by manual tracing or using semiautomatic border detection algorithms to trace the endocardium in cross-sectional images generated from the 3D data set segmentation. These contours can be combined together to generate a 3D shape that can be visualized as either a solid or a wireframe object used to create a 3D perspective. Wireframe reconstruction is used to generate 3D images of subsets of the entire data set in a cagelike picture.
Stereoscopic presentation of the left ventricle has been used to improve the visual assessment of ventricular shape as well as the appraisal of ventricular structures and the quantification of cardiac chamber volumes and function. However, surface rendering frequently fails to provide details of cardiac structures or textures. Solid and wireframe surface-rendering techniques can be combined to allow appreciation of the extent of cardiac structure motion (i.e., cardiac chamber volume changes during the cardiac cycle).
e
2D Tomographic Slices
The volumetric data set can be sliced or cropped to obtain multiple simultaneous 2D views of the same 3D structure. In this manner, the limitations of acoustic imaging with conventional 2D echocardiography can be overcome by 3D echocardiography, which allows the acquisition of different cutting planes from virtually any acoustic window. Indeed, it is possible to select unique 2D cutting planes (which may be difficult or virtually impossible to obtain with 2D transducer manipulation from standard windows) from a volumetric 3D data set and to display the corresponding 2D tomographic images in a cine loop format. For example, a cardiac chamber can be cut in true longitudinal or transverse planes, referred to as common long-axis or short-axis views.
Multiple slicing methods are available, such as arbitrary plane, simultaneous orthogonal (or arbitrary angle) slices, and parallel slice planes. The arbitrary plane cut allows the operator to orient the cutting plane in any direction, for optimal cropping of the cardiac structures of interest. The simultaneous orthogonal 2D slice mode consists of two or three 2D planes (coronal, sagittal, and transverse) displayed simultaneously. Finally, it is possible to obtain multiple 2D parallel tomographic slices with uniformly spaced 2D parallel slices.
These optimized cross-sectional planes of the heart allow accurate measurements of chamber dimensions and valve or septal defect areas as well as improved evaluation of the morphology and function of different structures with more objectivity and less operator dependency. The simultaneous orthogonal 2D slice mode provides multiple visualization of the same segment within a single cardiac cycle, which can be useful for ventricular function analysis as well as for wall motion assessment during stress echocardiography.
5
Management and Work Flow
Until 3D echocardiography is fully incorporated into daily clinical practice, protocols and techniques will remain focus oriented and vary according to disease process as well as institutional use. Currently, many laboratories perform full 2DE exams followed by focused 3DE studies. The reason for this inconvenient work flow was that the 2D image quality obtained with the 3D TTE probe was inferior to that of dedicated 2D TTE probes. Thus, the success of using 3DE in clinical practice depends on a practical work flow, which requires (1) a single transducer solution capable of 2D and 3D imaging, (2) accurate automated chamber quantification, and (3) automated display of standard 3DE or 2DE cut planes views from each window acquisition. With the latest generation of 3D TTE and TEE probes, the first requirement has been achieved, because the 2DE images obtained with these new transducers are comparable in quality with those obtained with dedicated 2D transducers. As well, multiple imaging ultrasound companies have developed or are in the process of developing software with automated chamber quantification and automated display of cut planes, which addresses the second and third requirements.
Beyond acquisition work flow, data management, which refers to the manner in which 3DE data are stored and recalled for analysis, also needs to be optimized. Currently, a 2DE exam requires on average 300 to 500 MB of storage space, whereas a combined 3DE and 2DE exam may require up to 1.5 GB of storage. These large data sets place a strain on the digital systems of laboratories not only with regard to transmission but also in terms of overall storage capacity. A Digital Imaging and Communications in Medicine standard for 3D echocardiography was approved in 2008, which called for the storage of Cartesian data sets without compression, which requires a large amount of digital storage space. Because of the storage requirements, this standard has not been widely adopted. Greater use of this standard and perhaps adoption of a standard with compression will ease 3DE data storage concerns.
6
3D Color Doppler Acquisition
a
TTE and TEE Data Acquisition
Similar to conventional 2D echocardiography, color Doppler superimposes flow information onto 3DE morphology. Three-dimensional color Doppler acquisition is performed using live 3D or multiple-beat full-volume acquisition. Although larger data volumes are achieved with multiple-beat full-volume color Doppler acquisition, it is limited by stitching artifacts. In contrast, live 3D color Doppler acquisition is not affected by stitching artifacts but is limited by smaller color Doppler volumes and lower frame rates. Although 3D color Doppler data acquisition is feasible with TTE and TEE examinations, 3D TEE acquisition currently provides significantly better color Doppler image quality and therefore is recommended for detailed color flow analysis. Similar to what occurs during non–color Doppler 3D data set acquisition, the size and location of the 3D color Doppler volumes should be carefully defined according to the flow region to be analyzed.
b
Cropping Methods
Color flow analysis includes (1) distal jets, (2) the proximal flow field of valvular flow regurgitation, and (3) flow through heart defects such as ventricular or atrial septal defects. Cropping of 3D color Doppler data sets follows the same principles as non–color Doppler data set cropping and is determined mainly by the analysis intended. For regurgitant jets, it is recommended to crop the 3D color Doppler data set to show two long-axis views of the jet: one with the narrowest and one with the broadest width of the jet. This display should also include a short-axis view of the jet at the level of the vena contracta ( Figures 5 and 6 ). Alternatively, color Doppler flow can be displayed using a multiple slice representation extracted from the 3D color Doppler data set, as shown in Figure 7 .
c
Orientation and Display
Understanding the orientation of color Doppler flow within the displayed views is clinically important. To help with the orientation, it is recommended to display the 3D color Doppler data in at least two different views with known orientation to each other as indicated by different colored cutting planes ( Figures 5 and 7 ). It is also recommended to display 3D color Doppler data together with characteristic anatomic 3D information using standard views.
d
Limitations
The limitations of 3DE color Doppler acquisition include poor spatial and temporal resolution, both expected to improve with the advancement of 3DE technology. Currently, live 3DE color Doppler acquisition is limited to small color Doppler volumes, usually with limited temporal resolution of 10 to 15 voxels/sec. Alternatively, multiple-beam full-volume acquisition of color Doppler providing larger color Doppler volumes and volume rates (up to 40 voxels/sec) are limited by stitching artifacts, resulting in significant displacement between different subvolumes ( Figure 3 , bottom ).
7
Transthoracic 3DE Examination Protocol
Three-dimensional TTE full-volume acquisition mode can accommodate most of the entire heart structures within a single 3D data set. However, with existing technology, the decreases in both spatial-temporal resolution and penetration that would result from enlarging the volume angle to acquire the entire heart from a single acoustic window makes this impractical. To overcome these limitations, 3DE data sets “should be” acquired from multiple transthoracic transducer positions.
In clinical practice, two protocols have been used: (1) focused examination and (2) complete examination. A focused 3DE examination usually consists of relatively few 3DE data sets acquired to complement a complete 2D study. Some examples of focused 3DE examinations are (1) acquisition of a gated 3DE full-volume data set from the apical window to quantify LV volumes, LV ejection fraction, and LV shape and to evaluate for LV dyssynchrony in patients with heart failure; (2) data sets acquired from both the parasternal and apical approaches to visualize the mitral valve apparatus with the aim of measuring orifice area in a patient with mitral stenosis; and (3) 3D zoom mode acquisition, with high density from the parasternal window to visualize the aortic valve in a patient with suspected bicuspid valve. For a focused exam, start with 2D imaging to localize the structure of interest, then switch to live 3DE imaging to check if the structure of interest is encompassed within the volume of interest, and then obtain a 3D acquisition in the full-volume or zoom mode. If the patient is unable to hold the respiration during a gated multiple-beat acquisition or if significant rhythm disturbances are present, use single-beat full-volume acquisition (if available) or use narrow-angled 3D acquisition mode. Last, select the highest resolution option that accommodates the volume of interest.
A complete 3D TTE exam requires multiple acquisitions from the parasternal, apical, subcostal, and suprasternal transducer positions. Table 2 lists the 2D TTE views from which 3DE data sets should be acquired. Because the volume-rendered 3D data set can be cropped to display a variety of intracardiac structures by choosing different cut planes as an alternative to “view” (referred to heart’s orientation to the body axis), “anatomic planes” (referred to the heart itself) can be used to describe image orientation. The most frequently used cropping planes are (1) the transverse plane , a horizontal plane that runs perpendicular to the long axis of the body dividing the heart into superior and inferior segments; (2) the sagittal plane , a vertical plane that divides the heart into right and left segments; and (3) the coronal plane , a vertical plane that divides the heart into anterior and posterior segments ( Figure 8 ).
Protocol For Three-Dimensional Transthoracic Echocardiography | ||
---|---|---|
Aortic Valve | Left Ventricle/Right Ventricle | Pulmonic Valve |
Parasternal long-axis view with and without color (narrow angle and zoomed acquisitions) | Apical four-chamber view (narrow and wide angle acquisition) Please note that the image must be tilted to place the right ventricle in the center of the image for right ventricular acquisition | Parasternal right ventricular outflow tract view with and without color (narrow angle and zoomed acquisitions) |
Mitral Valve | Interatrial and Interventricular Septum | Tricuspid Valve |
---|---|---|
Parasternal long-axis view with and without color (narrow angle and zoomed acquisitions) | Apical four-chamber view (narrow angle and zoomed acquisitions) | Apical four-chamber view with and without color (narrow angle and zoomed acquisitions) |
Apical four-chamber view with and without color (narrow angle and zoomed acquisitions) | Parasternal right ventricular inflow view with and without color (narrow angle and zoomed acquisitions) |
8
Transesophageal 3DE Examination Protocol
A comprehensive 3DE examination using the matrix TEE transducer usually starts with real-time imaging modes such as live and narrow-angled acquisition. However, the gated 3DE modes, including 3D color flow Doppler, should also be used whenever ECG and respiration gating requirements are permissible, to take advantage of the improved spatial and temporal resolution of these wide-angled acquisitions. Three-dimensional TEE data sets acquired using a matrix array include both the area and depth of the imaging plane, thereby requiring less probe manipulation for data acquisition compared with a standard 2D TEE examination. Furthermore, unique en face 3DE views with infinite real-time rotational and cropping plane capabilities, as well as offline quantitative analyses, should result in accurate diagnoses and ultimately improved clinical decision making. Although a systematic approach to performing a comprehensive 3D TEE examination is recommended, it is recognized that not all views may be optimally obtained in all patients and that additional unconventional views may be required to obtain additional detailed information in patients with complex pathologies.
Initially, a real-time 3DE and a subsequent gated 3DE data set should be obtained from the midesophageal views to determine the overall function of the left and right ventricles and to identify structural valve abnormalities. Table 3 describes the recommended views to obtain 3D images of cardiac structures using transesophageal echocardiography. Table 4 demonstrates how to display 3D TEE images of the cardiac valves from the original 2D TEE views. Offline analyses of the gated 3DE data set acquired from the midesophageal five-chamber view can be performed to obtain quantitative measures of LV global and regional function.
Protocol For Three-Dimensional Transesophageal Echocardiography | ||
---|---|---|
Aortic Valve | Left Ventricle/Right Ventricle | Pulmonic Valve |
60° mid-esophageal, short-axis view with and without color (zoomed or full-volume acquisition) | Left ventricle – 0° to 120° mid-esophageal views encompassing the entire ventricle (full-volume acquisition) | 90° high-esophageal view with and without color (zoomed acquisition) |
120° mid-esophageal, long-axis view with and without color (zoomed or full-volume acquisition) | Right ventricle – 0° to 120° mid-esophageal views with the right ventricle tilted to be in the center of the image (full-volume acquisition) | 120° mid-esophageal, 3-chamber view with and without color (zoomed acquisition) |
Mitral Valve | Interatrial Septum | Tricuspid Valve |
---|---|---|
0° to 120° mid-esophageal views with and without color (zoomed acquisition) | 0° with the probe rotated to the interatrial septum (zoomed or full- volume acquisition) | 0° to 30° mid-esophageal, 4-chamber view with and without color (zoomed acquisition) |
40° transgastric view with anteflexion with or without color (zoomed acquisition) |
9
Assessment of the LV
Accurate and reproducible quantitative assessment of LV size and function are pivotal for diagnosis, treatment, and prediction of prognosis of structural heart diseases. In this regard, the most important contribution of 3D echocardiography may be in LV quantification. Cumbersome acquisition methods and lack of user-friendly analysis software initially precluded widespread use of 3D echocardiography, but the advent of matrix transducers, together with impressive improvements in semiautomated volumetric analysis, has allowed 3D echocardiography to evolve from a complicated and time-consuming research tool into a simple and fast imaging modality ready for everyday clinical use.
a
Anatomy and Limitations of 2DE Assessment
The purpose of 3D imaging of the left ventricle is to provide volume and ejection fraction measurements independent of geometric assumptions regarding LV shape. The landmarks used for this process are the mitral annulus and LV apex, which are used to initiate edge detection by semiautomated quantification software. Other anatomic features of importance are the LV trabeculae and papillary muscles, which should be included within the LV cavity for the calculation of LV volumes. The trabeculae are small structures that are often poorly visualized with 3DE imaging, and the use of LV opacification with contrast is the best way to ensure that they are incorporated within the LV cavity.
The assessment of wall motion requires a frame of reference to allocate segments. The 17-segment model distinguishes six segments (inferoseptal, anteroseptal, anterior, lateral, inferolateral, inferior) in the base and mid left ventricle, four segments (septal, anterior, lateral, inferior) in the apex, and the apical cap. This segmentation is based on the mitral annulus, papillary muscles, and apical portion of the LV cavity to define the planes in the longitudinal dimension. In the transverse dimension, the segments comprise 60° arcs, starting at the midpoint of the septum, which itself is defined by the anterior and posterior RV insertion points of the septum. A little more problematic is the definition of regional LV volume, currently defined in most programs by the space between the endocardial border and a centerline through the LV cavity. This virtual landmark may shift with alterations of LV mass and remodeling after myocardial infarction, leading to underestimation of serial regional volume changes. The use of an externally defined frame of reference could overcome this problem, but it is unclear how this can be readily achieved.
LV structural changes that can be identified with 3D echocardiography include ventricular septal defects and masses such as LV thrombi or tumors. The sites of these are usually described by reference to the relevant landmarks (mitral annulus, LV outflow tract, and apex) or segmentation.
Despite the utility and established role of 2D echocardiography to assess LV function, it has a number of important limitations for LV imaging, including foreshortening, malrotation, and angulation. Because of the geometric assumptions of 2D echocardiography, volumetric measurements may be inaccurate if the acquisition of 2D images is suboptimal. Likewise, appropriate interpretation of regional LV responses to stress requires all vascular territories to be imaged and for analogous segments to be displayed at rest and stress.
As with its use in other situations, there are fundamentally two approaches to the application of 3D echocardiography. The first involves the use of a full-volume data set to create standard 2D images in which the cut planes are optimized to ensure that they are “on axis”; this is the strategy used for segmental wall motion assessment and tracing of LV borders for volume calculations. In segmental imaging, there are benefits of obtaining orthogonal views to confirm wall motion abnormalities in any segment. The second is a display of rendered images that provides a 3D impression of a structure, such as might be used for the assessment of LV mass or thrombus.
These benefits are obtained at the cost of technical shortcomings of 3D echocardiography. The probe footprint of most 3D transthoracic transducers is large, which contributes to difficulty in imaging the anterior and lateral walls because of interference from ribs. Recently, newer 3D transthoracic transducers have been developed with smaller footprints to overcome these limitations. Second, there is lower line density and therefore lower spatial resolution of 3D echocardiography, which may be partly readdressed with the use of LV opacification with contrast. Third, 3D echocardiography has lower temporal resolution than 2D echocardiography because of the lower volume rate that is attainable with 3D echocardiography. This can be addressed by narrowing the 3D sector and stitching multiple subvolumes, but at the risk of creating stitching artifacts and with specific limitations in irregular rhythms or inability to hold the breath.
b
Data Acquisition and Cropping
Data Acquisition
A 3DE acquisition of the entire left ventricle generally takes <10 sec. Although all standard acquisition windows for echocardiography are available and useful for 3D acquisitions, the ideal and generally preferred approach for the acquisition of a full-volume LV data set is the apical one ( Tables 2, 3, and 5 ). Depending on the shape of the heart and its position within the chest, a more off-axis position may be appropriate to ensure the acquisition of the entire left ventricle. To guarantee optimal image quality, transducer frequency and overall gain should be adjusted accordingly. The best sequence for image optimization is to follow the 2D images with real-time 3D images—although there is limited value in real-time imaging of the left ventricle (except for structural changes such as mass or thrombus), this step is of value to optimize gain settings—which should be typically higher than those used for 2D echocardiography. Acquisition of the full-volume data set can be guided by a split-screen display of orthogonal views, which can itself be used for simultaneous imaging in two or three planes. The full-volume acquisition should be made during a breath hold to minimize the risk for breathing (stitch) artifacts. As discussed above, contrast LV opacification is often of value.
c
Orientation and Display
There is no general agreement on how the imaging planes should be displayed. The proposed “apex-down” 3D display has not been widely adapted for LV imaging, perhaps because LV imaging is “3D-guided 2D.” The preference of the writing group is to orient images so that right-sided structures are on the left-hand side and the apex is up ( Figure 9 ).
d
Analysis Methods
Volume rendering is of primary value for demonstration of structural abnormalities. Within the left ventricle, these might include thrombi, masses and septal defects. This approach is of limited value for the quantification of LV function.
Surface rendering is of primary value for global and regional functional measurements, including 3D echocardiography–guided 2D imaging for measurement of LV volume, ejection fraction and mass. Most vendors offer software packages for both online and offline quantitative analysis of the left ventricle. Typically, this process involves segmentation of the 3DE data set into several equiangular 2D longitudinal planes after initialization of a few anatomic landmarks, such as the mitral annulus and apex, in several conventional 2D planes. If necessary, manual corrections to the endocardial borders can be performed, after which a semiautomated blood endocardial interface detection algorithm allows the calculation of cavity contours and display of their changes during the cardiac cycle providing a volume-versus-time curve ( Figure 10 ). A surface-rendered cavity cast of the left ventricle is then constructed, from which LV volume is computed without geometric assumptions, directly from voxel counts.
Wireframe models are effective for defining sections of the left ventricle in position and time. These 3DE data can be used to assess LV synchrony, regional strain, curvature, and wall stress. However, these steps are computationally intensive and are not performed in routine practice.
The analysis of regional function is more complex to communicate than global function or even shape. One option is a polar map display incorporating wall motion scoring; a similar and more dynamic process may be illustrated using contraction front mapping ( Figure 11 ), which illustrates the spatial distribution of contraction and relaxation.
e
Clinical Validation and Application
LV Structural Abnormalities (e.g., Thrombus, Ventricular Septal Defect)
These are assessed using visual assessment and 3DE color flow mapping.
Global LV Functional Measurements
These include volumes, ejection fraction, LV shape, and regional and global strain. Of these, LV volumes and ejection fraction are the closest to clinical application. This measurement of LV volume and function is rapid, more accurate and reproducible than with 2DE, and has an accuracy that is similar to magnetic resonance imaging, although the variability may be higher as a result of varying image quality and operator expertise. The availability of LV cavity shape allows the extraction of additional quantitative information in patients with LV dysfunction (e.g., the 3D sphericity index). The assessment of 2D global strain is an interesting potential marker of global function; whether this measurement can be reliably assessed with 3D imaging remains undefined at present.
Despite the high correlation with magnetic resonance imaging as the reference technique, several studies using both manual and semiautomated contour detection have shown significant underestimation of 3D echocardiography–derived LV volumes. The potential reasons for the underestimation are numerous, but systematic underestimation of LV volumes by 3D echocardiography compared with magnetic resonance imaging may be largely explained because 3D echocardiography, unlike magnetic resonance imaging, cannot consistently differentiate between the myocardium and the trabeculae. To minimize intertechnique differences, tracing the endocardium to exclude trabeculae in the LV cavity is recommended for 3D echocardiography. As well, one-beat acquisitions may not successfully capture true end-systole, because of the reduced temporal resolution. This will lead to inaccurate end-systolic volume calculations and ejection fraction measurements.
The reproducibility of LV volume and function measurements by 3D echocardiography has been assessed in multiple studies. Most of these studies were part of larger studies in which series of patients were analyzed twice by one observer and by a second observer. Less variation is reported than with 2D echocardiography. The best reproducibility was obtained in studies that selected patients on the basis of good image quality. Differences between observers are less likely to be of technical origin. Although some differences have been found to be statistically significant between different baseline settings with different semiautomated endocardial contour tracing algorithms, they do not seem clinically relevant. The normal values of LV end-diastolic and end-systolic volume have not been established by gender and body size and so are not provided in this document.
LV Mass
More user interaction is required to identify the epicardium for the calculation of LV mass. Despite a slight overestimation of LV mass by 3D echocardiography in comparison with magnetic resonance imaging measurements, the accuracy of 3D echocardiography is similar to that of magnetic resonance imaging in most patients. However, there are wide limits of agreement, reflecting a number of issues. First, the use of innermost versus outermost endocardial contour tracing might explain measurement differences, as LV mass measurement increases with the inclusion of trabeculae into the LV wall. Second, LV mass measurement also relies on accurate visualization and tracing of the epicardial contour, which is even more challenging than endocardial tracing. Takeuchi et al. and Pouleur et al. used echocardiographic analysis software that semiautomatically detected the endocardial surface and then calculated LV mass by arbitrarily adding 8.8 mm of wall thickness to the endocardial surface. Third, analysis of short-axis magnetic resonance images and long-axis 3DE images is distinctly different.
LV Dyssynchrony
For the analysis of LV dyssynchrony, individual LV segmental volumes are plotted versus time throughout the cardiac cycle. These plots allow measurement of temporal differences in segmental time to minimum volume. Regional minimal volume (i.e., maximal contraction) normally occurs at the same time in ventricular systole for all segments. In a left ventricle with dyssynchrony, there is dispersion in the timing of regional segments reaching minimal volume as the diseased segments achieve minimal volume later in systole ( Figures 10 and 11 ). The systolic dyssynchrony index (SDI) is calculated as the standard deviation of regional ejection times (time to regional minimal volume). Parametric images using color schemes representing timing differences in segmental contraction can be displayed in a “bull’s-eye” format, which is a practical tool for identifying and localizing areas of dyssynchrony.
The literature on 3DE dyssynchrony has focused on three major areas: (1) description of reference values and reproducibility of SDI in normal subjects and different patient subsets, (2) comparison of 3DE measurements of SDI with those using conventional methods (i.e., tissue Doppler), and (3) prediction of responses to cardiac resynchronization therapy (CRT) using 3D echocardiography–derived LV SDI.
Doppler tissue imaging has high temporal resolution but only provides information on longitudinal systolic myocardial contraction of the mid and basal segments, frequently with low reproducibility. In contrast, 3D echocardiography evaluates all LV segments simultaneously. Three-dimensional echocardiography–derived LV SDI was described as highly predictive of response to CRT at 48 hours, 6 months, and 1 year of follow-up. Benefits from CRT have been defined as a ≥15% reduction in LV end-systolic volume at follow-up, which can also readily be measured using 3D echocardiography.
Finally, the importance of optimal LV pacing lead position was emphasized in a 3DE study in which the responses to CRT were compared between patients with the LV pacing lead positioned at the site of maximal mechanical delay and those in whom the pacing lead was positioned distal to that site. LV function, reverse remodeling, and peak oxygen consumption were significantly improved in patients with optimal LV pacing lead position guided by 3D echocardiography, whereas the opposite occurred with increasing distance between the optimal and the actual pacing site. Currently, because these data come from small, single-center, nonrandomized studies, patients should not be selected for CRT on the basis of 3DE parameters until more data become available.
f
Future Perspectives
A large amount of evidence suggests that in the presence of adequate image quality, LV volumes and functional measurements by 3D echocardiography have closer limits of agreement with cardiac magnetic resonance measurements and better reproducibility than 2D echocardiography, making it the modality of choice for the everyday clinical evaluation of LV volumes and ejection fraction. Furthermore, LV dyssynchrony assessment by 3D echocardiography might play a valuable role in the selection of patients for CRT through the prediction of response but also prove to be useful for the optimization of LV lead placement. Other developments, such as 3D strain measurement and LV shape analysis, show great potential to become future clinical applications. Future advancements in hardware will facilitate the acquisition of wider angle pyramidal data with higher spatial and temporal resolution in a single cardiac cycle. To continue to enhance the clinical applicability of 3DE imaging, further improvements in automatic quantitative analysis software that will enable fast online measurements that are accurate and reproducible are required.
Currently, 3D TTE and TEE assessment of LV volumes and ejection fraction is recommended over the use of 2D echocardiography, as it has been clearly demonstrated to provide more accurate and reproducible measurements.
10
Assessment of the RV
a
Anatomy and Limitations of 2DE Assessment
The right ventricle is composed of three anatomic and functional subunits, which extend from (1) the tricuspid valve (TV) annulus to the proximal os infundibulum, (2) the RV body to the apex, and (3) the RV outflow tract to the pulmonary valve. This divides the RV cavity into three sections: inlet, apical trabecular, and outlet, respectively. The musculature of the right ventricle extends from the atrioventricular to the ventriculoarterial junctions. The right ventricle is highly trabeculated, with several muscle bands, including the septoparietal trabeculations and the moderator band. From a functional point of view and because of the orientation of the RV fibers, global assessment of the right ventricle is difficult, with the two main sections contracting perpendicular to each other: the proximal (RV inflow) longitudinally and the distal (RV outflow) circumferentially.
Because of the peculiar RV morphology and function, 2D echocardiography has several limitations in the evaluation of the right ventricle, which can be readily overcome by a 3DE gated wide-angled acquisition, which enables complete assessment of its geometry, volumes, and ejection fraction ( Figure 12 and 13 ), displaying the surfaces of the entire right ventricle including the inflow, apex, and outflow tracts.
b
Data Acquisition
Several methods and software packages have been used to evaluate the right ventricle. Three-dimensional data are acquired in a full-volume data set from the four-chamber apical view adapted to include the entire right ventricle ( Tables 2 and 3 ). Three-dimensional echocardiographic data sets are typically digitally stored and then postprocessed offline. On-cart dedicated RV analysis software packages will be soon available, further facilitating the use of these measurements in clinical practice. Current RV analysis software displays 2D cut planes of the RV sagittal, four-chamber, and coronal views obtained from the full-volume 3DE data set.
c
Orientation and Display
The anatomy and pathology of the TV and the right ventricle are best visualized using volume-rendered images ( Table 5 ). When performing volumetric analysis using semiautomated border tracking software data, the right ventricle may be displayed as a wireframe or surface-rendered cast. Studies describing quantitative changes in tricuspid annular enlargement and leaflet tenting have used the wireframe method. Cropping tools can be applied to 3DE data sets to visualize the RV inflow and outflow tracts. The TV can be displayed from both the right atrial and RV perspectives. The right atrial view of the TV should be modified to a surgeon’s orientation, as seen in Figure 14 . The TV should be displayed with the septal leaflet at the 6 o’clock position, irrespective of perspective. The right atrium and right ventricle can be visualized using multiple cut planes. A variety of axial cuts at the apex, mid, and base of the right ventricle can be obtained using the long axis of the left ventricle. In Figure 13 , an axial cut plane of the right ventricle depicts a view of the TV and RV outflow tract on the left. Longitudinal cut planes can also demonstrate the right ventricle from a typical four-chamber view, coronal view, and RV inflow view. The position of these cut planes is represented on the axial view. The four-chamber view of the right ventricle demonstrates the RV free wall and septum. The coronal cut plane demonstrates the right atrium, TV (septal and posterior leaflets), RV inflow and outflow, and pulmonic valve. The RV inflow view shows the right atrium, anterior and posterior TV leaflets, and interventricular septum.
d
Analysis Methods
The current methods used to quantify RV geometry and functions include the method of disks, a rotational approach, and most recently a volumetric semiautomated border detection approach. Two of these methods are currently commercially available and are shown in Figure 15 .
Method of Disks and Other Methods
A variety of options for offline 3DE reconstruction of the right ventricle exist. After acquisition and automatic display of the RV end-diastolic and end-systolic frames, the operator, in the axial plane, traces a contour of the endocardial border. These traced contours generate disks of fixed height (generally 10 mm) but of varying lengths and widths, as visualized in the other RV orthogonal views. The volume of the RV cavity is computed by adding the known areas of the axial traces obtained 10 mm apart (i.e., disk summation). The number of disks required to cover the entire right ventricle from base to apex varies from seven to eight depending on RV size.
Recently available software calculates RV volumes from end-diastolic and end-systolic endocardial border tracings of sagittal (to outline the TV in the best possible view), four-chamber (to outline the apex), and coronal (to outline the RV outflow tract) 3D echocardiography–derived cross-sectional planes. The operator frequently needs to manually adjust the traced contours in each frame before reconstruction and quantitative analysis. Trabeculations are generally included in the endocardial rim, but the apical component of the moderator band is excluded from the cavity. The RV volumes are calculated by summation of the volumes for each slice through the complete data set. Each volume data set is imported into the application and manipulated by rotating, angulating, and slicing in any of the three displayed orthogonal planes. This software analysis, which uses a semiautomated border detection algorithm with manual correction options, was validated using in vitro models as well as in vivo using cardiac magnetic resonance as the gold standard.
Volumetric Display
The different software packages create a surface-rendering cast of the right ventricle. The end-diastolic and end-systolic volumes as well as RV ejection fraction are measured and automatically displayed. Segmental analysis of the three main sections of the right ventricle (inlet, apex, and outflow segments) may be performed. Curves of global and regional RV function may be generated and analyzed.
e
Clinical Validation and Application
Data on RV volumes and function are of diagnostic and prognostic importance in a variety of cardiac diseases, including valve disease, congenital heart disease, pulmonary hypertension, and heart failure. Three-dimensional echocardiography allows the quantification of volumes and function in normal subjects and patients, thereby allowing identification of patients with different degrees of severity of RV dilatation and dysfunction. Several clinical studies have shown a good correlation between cardiac magnetic resonance and 3DE volumes and ejection fraction of the right ventricle in selected populations, with the majority of studies showing a slight underestimation of volumes compared with the reference technique. Differences in RV volumes have been demonstrated between men (129 ± 25 mL) and women (102 ± 33 mL), but adjusting to lean body mass (but not to body surface area or height) eliminated this difference. The use of 3D transthoracic echocardiography has been validated in patients with pulmonary regurgitation, secundum atrial septal defects, tetralogy of Fallot repair, Ebstein’s anomaly, and RV cardiomyopathy. The feasibility and utility of 3D transthoracic echocardiography for guidance of RV endomyocardial biopsies in children has also been demonstrated.
Assessment of RV function is of great interest in cardiovascular surgery, because right-sided heart failure is one of the most frequent causes of morbidity and mortality after valvular and congenital surgery, coronary artery bypass, and heart transplantation. This highlights the importance of an accurate preoperative assessment of the right ventricle to improve risk stratification and early and precise postoperative follow-up to optimize treatment. In this regard, 2DE and Doppler parameters (tricuspid annular plane systolic excursion, tissue Doppler imaging of the annulus) have several limitations, particularly in postoperative follow-up. The evaluation of RV volumes and ejection fraction using 3D echocardiography overcomes many of the limitations of 2DE methods.
Currently, 3DE assessment of RV volumes and ejection fraction shows great promise. However, routine clinical use is limited by the need for excellent quality transthoracic data sets for accurate analysis with software packages .
11
Mitral Apparatus
a
Anatomy and Limitations of 2DE Assessment
The mitral apparatus is formed from the hyperbolic paraboloid (i.e., saddle) shaped annulus, the multiscalloped and indented leaflets connected by opposing anterolateral and posteromedial commissures, the subvalvular apparatus composed of a highly variable chordae tendineae arrangement with dual papillary muscles, and the LV wall attachments. Function of this apparatus requires an intricate coordination between multiple anatomic components, each of which has a unique functional geometry. Three-dimensional echocardiographic imaging modalities are ideal for interrogating the anatomy and function of each of the individual components of the mitral apparatus.
Mitral Leaflets
The anterior mitral valve leaflet has the larger radial surface and is attached to about one third of the annular circumference. The posterior leaflet has a larger circumferential attachment (two-thirds of the annulus) and is quadrangular is shape. Both leaflets are segmented into three individual scallops: A1, A2, and A3, the anterior, and P1, P2, P3, the posterior (from left to right, respectively; Figure 16 ). Leaflet segmentation is particularly useful to precisely localize prolapsing segments and anatomic lesions of the mitral valve. The coaptation line has an upward concavity and can be checked for visible leaks while in the closed position. The anterior mitral valve leaflet is in continuity with the left and noncoronary cusps of the aortic valve (aortic-mitral curtain ), as seen from the LV perspective in a modified oblique view of the atrioventricular plane ( Figure 16 ).
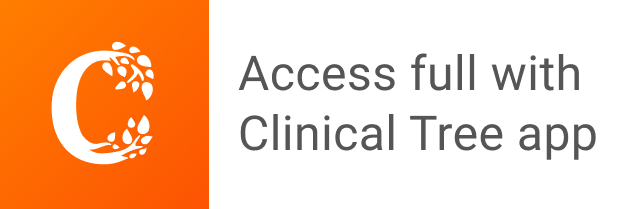