, Dilip R. Karnad2 and Snehal Kothari3
(1)
Cardiac Safety Services Quintiles, Durham, North Carolina, USA
(2)
Research Team, Cardiac Safety Services Quintiles, Mumbai, India
(3)
Cardiac Safety Services Global Head, Cardiac Safety Center of Excellence Quintiles, Mumbai, India
Drug-induced biological activity is generated in three-dimensional space: biological structures acting as drug receptors and the drug molecules that interact with them are three-dimensional entities.
2.1 Introduction
Drugs exert their therapeutic benefit by interacting with a target biological structure, generating a cascade of beneficial biological signaling and consequently changing a patient’s biology for the better. Unfortunately, drugs can also lead to undesirable biological consequences, including adverse cardiovascular events. Before discussing the nature of these undesirable consequences and the methodologies for their prospective exclusion in subsequent chapters, it is appropriate to become familiar with the biological structures and systems activated by a drug. It is also useful to become familiar with the nature of the structure of a drug. This chapter therefore commences with a conceptual discussion of the structure of small-molecule drugs. It then discusses biological systems and the nature of interactions between biological structures and small-molecule drugs. Finally, it discusses drugs that are macromolecules: these discussions follow the introduction to biological systems since the nomenclature introduced there facilitates their description.
Biological considerations in this chapter are addressed from a pragmatic perspective and tailored specifically to suit current needs. In keeping with our goal of writing a self-contained book on cardiovascular safety, our discussions have been geared towards readers who will benefit most from them: readers who are already well versed in basic biology may prefer to progress directly to the next chapter.
2.2 Small-Molecule Drugs: New Molecular Entities and Drug Molecules
A molecule is the essence of a substance, the smallest unit of that substance that still retains the substance’s chemical identity. The atoms within a molecule can be conceptualized as being grouped into various molecular components called functional groups. A common functional group in acidic molecules, for example, is the carboxylic acid group, represented in the language of atoms as “COOH.” This functional group consists of a carbon atom, two oxygen atoms, and a hydrogen atom. Functional groups determine the chemical and physical properties of molecules.
Drug-like molecules possess certain characteristics. For example, they have a relatively low molecular weight and possess one or more functional groups that are held together on a structural framework or backbone. This backbone needs to be relatively rigid to ensure that the shape of the molecule does not alter too much. The functional groups are therefore positioned in three-dimensional space in a specific geometrical array and are available to interact with drug receptors within the body. The term drug-like molecule refers to a molecule that could theoretically be a match for a site on a receptor: once it is known that the molecule will bind (interact) with a specific receptor site, the term drug molecule is used.
A drug molecule can be regarded as a “collection of molecular fragments held in a three-dimensional arrangement that determines and defines all of the properties of the drug molecule” (Nogrady and Weaver 2005). These properties include physiochemical, shape and stereochemical, and electronic properties. They are important in determining whether or not a drug molecule that is administered to an individual will actually reach the microenvironment of the drug target and, if it does, whether or not it will interact with the drug target successfully.
Nogrady and Weaver (2005) discussed the influences of these properties. Physiochemical properties impact a drug’s solubility and pharmacokinetic characteristics, influencing the drug’s ability to reach the region of the body in which the drug target is located, which can be a long way from the site of the drug’s administration (oral administration is common, but by no means the only way a drug can be administered). Shape and stereochemical properties affect the pharmacodynamic phase of drug action and influence the drug’s interaction with its target receptor. These properties describe the structural arrangement of the drug molecule’s constituent atoms and influence the molecule’s final approach towards the target receptor. Electronic properties also affect the pharmacodynamic phase of drug action. The electronic properties of a molecule are governed by the distribution of electrons within the molecule and give each region of a molecule a positive, negative, or neutral charge. These properties determine the exact nature of the binding interaction that occurs between the drug and its target receptor and the degree to which the interaction is energetically favorable. The energetic exchange that occurs between the drug molecule and the receptor determines the strength of the biological signal that is generated. It is this signal that governs the pharmacological (biological) effects of the drug.
2.2.1 Pharmacophores and Toxicophores
Wermuth (2006) discussed the terms pharmacophore and toxicophore. A pharmacophore is a concept used to account for the common molecular interaction capacities of a group of compounds towards their target receptor. It can be considered as the highest common denominator of a group of molecules exhibiting a similar pharmacological profile and which are recognized by the same target receptor. It describes the essential (steric and electronic) function-determining points necessary for an optimal interaction with a relevant target receptor. Once the drug molecule enters the microenvironment of the target receptor, it is necessary that the geometry of the molecule precisely matches the geometry of the receptor site on the target receptor molecule. The pharmacophore therefore needs to be spatially and geometrically positioned consistently as the drug molecule approaches the target receptor.
At this point, it is worth introducing stereoisomers. These are isomeric molecules that have the same molecular formula and hence the same sequence of bonded atoms. However, they differ in the three-dimensional orientation of their constituent atoms. For example, consider the example of the antiarrhythmic drug sotalol. Both d-sotalol and I-sotalol isomers affect I Kr that influences cardiac repolarization, but only the I-isomer has beta-blocking effect. Another example is warfarin. The S-isomer is three to five times more potent than the R-isomer, and they are metabolized by different cytochrome pathways, an influence that contributes to variable response and toxicity between batches of the drug and ethnic populations.
In a similar manner, and of greater relevance for this book’s discussions, a toxicophore is conceptualized as an assembly of geometrical and electronic features of a different functional group of atoms in the drug molecule that interacts with a nontarget receptor and elicits an unwanted biological response or side effect. There is no meaningful structural or molecular difference per se between a pharmacophore and a toxicophore: the names are determined by intent, with a pharmacophore intended to interact with the target receptor, and a toxicophore unintentionally interacting with an off-target receptor and leading to an undesirable effect. (There are instances where a drug being developed for use in particular disease has demonstrated unanticipated off-target effects that have serendipitously been desirable effects for another disease, and the drug became marketed for that indication.)
2.2.2 Drug Discovery and Drug Design
Traditionally, the terms drug discovery, nonclinical development, and clinical development have been used to describe the process of research conducted to bring a new drug to marketing approval. Along with postmarketing surveillance, they are components of lifecycle drug development. Over the course of human history, many pharmacologically active agents were naturally occurring chemical substances, and discoveries of their medicinally beneficial properties were serendipitous rather than deliberate. As the pharmaceutical industry was formed, the process of drug discovery involved the evaluation of many molecules in a systematic manner to determine those with interesting pharmacological characteristics. It was quite possible that hundreds (if not thousands) of potential drug candidate molecules would be tested for pharmacodynamic action in what was a very laborious process.
More recently, as biomedical science has advanced rapidly and knowledge of molecular chemistry and molecular biology has increased dramatically, small-molecule drug discovery has become more driven by technology. The starting point in recent small-molecule drug development is often knowledge of the molecular structure of the drug’s target receptor. Given this knowledge, molecular technologies are utilized to discover a drug whose molecular structure is appropriate to facilitate the desired pharmacological effect. Additionally, the term drug design has become common. Drug design is concerned with modifying the structure of an existing chemical molecule in specific ways, including modification of its pharmacokinetic profile or synthesizing a related new chemical molecule specifically for its pharmacological benefit.
As Nogrady and Weaver (2005) observed, the interdisciplinary science of medicinal chemistry provides “a molecular bridge between the basic science of biology and the clinical science of medicine.” It focuses on the discovery/design of new molecular entities, their optimization, and their development as useful drug molecules for the treatment of disease. The authors also provided a comprehensive definition of a useful drug. A useful drug molecule has the following properties: it is acceptably safe; it is efficacious for the disease or clinical condition of concern; it is well tolerated; it can be manufactured in sufficiently large quantities by processes that can comply with all necessary regulatory oversight and that are financially viable for the sponsor; it can successfully navigate all necessary regulatory oversight, including those that govern nonclinical investigations and clinical trials, and be approved by regulatory agencies for marketing; and it can be successfully marketed and therefore be prescribed by clinicians (Nogrady and Weaver 2005).
With regard to the optimization of new molecular entities, there are three considerations: optimizing therapeutic benefit, minimizing toxicity resulting from the molecule’s interaction with nontarget receptors, and, at the same time, optimizing pharmacokinetic properties such that the drug reaches its target receptor satisfactorily. Contemporary drugs are typically foreign substances, or xenobiotics, and the body therefore attempts to break them down as efficiently as possible. Therefore, regular short-term (perhaps one tablet a day for 7–10 days for an antibiotic) and long-term (perhaps one tablet a day for many years for an antihypertensive medication) administration is typically necessary.
Modern computing techniques are increasingly being used in drug discovery and design. Two recent trends have facilitated this paradigm shift. First, the phenomenal growth in information from molecular biological studies provides the pharmaceutical industry with tremendous opportunities to capitalize on this information in the development of new drugs. This includes a knowledge of how genetic material codes for the production of proteins, knowledge of the structure and function of proteins, how proteins create metabolic pathways, and how environmental factors affect the phenotypic expression of a person’s genotype to create a unique individual human being (even in the cases of identical twins and other identical multiple births) with a unique set of metabolic pathways.
The second trend is a tremendous advancement in computing systems (often sophisticated networks, or clusters, of relatively small computers rather than hugely powerful individual machines). The combination of advances in these areas has facilitated the development of bioinformatics and cheminformatics, highly computational fields that deal with storing and communicating the ever-increasing amount of molecular biological and chemical data available.
Historically, in vitro and in vivo testing has been the mainstay of evaluating a drug molecule’s action before proceeding to clinical trials. The advent of tremendous computing power has changed many aspects of drug discovery and development, facilitating another approach known as in silico testing. Before a molecule is synthesized, extensive computer modeling takes place in an attempt to identify a molecular structure that would likely have a high probability of achieving the desired interaction with a target receptor. In silico development focuses on many aspects of the molecule, including its ability to reach the region of the drug receptor, its ability to approach and dock (bind) with the receptor, and the precise nature of the binding interaction with the target receptor. Of considerable relevance to later discussions in this book is that this field is also concerned with binding interactions with nontarget receptors.
Knowledge of the structure of the receptor macromolecule now permits research scientists to adapt the approaches of computer-assisted design to create the field of computer-assisted molecular design (CAMD). In combination with the disciplines of bioinformatics and cheminformatics, it has become possible to design lead compounds from scratch that are probabilistically well suited for further development and optimization. CAMD facilitates in silico three-dimensional docking experiments, i.e., simulations of potential drug molecules docking with receptors. The results of these experiments can identify a potentially safe and efficacious drug molecule relatively more easily, less expensively, and very much more quickly than other approaches in drug discovery.
As a lead compound is optimized, enhancing the features of the pharmacophore to elicit a more energetic therapeutic interaction with the receptor is desirable, as is modifying toxicophores to lessen or eliminate side effects. In addition to these pharmacodynamic and toxicodynamic considerations, pharmacokinetic considerations need to be addressed. The goal in this case is to modify the metabophore (the functional group of atoms in the drug molecule responsible for its pharmacokinetic properties) where possible to minimize the drug’s metabolism in the liver and its rapid excretion by the kidneys, thereby giving the molecule a greater chance to reach its target receptor and exert its desired pharmacodynamic effects.
2.2.3 Structural Molecular Engineering: A Case Study
In combination with in silico modeling, structural molecular engineering offers the possibility of creating an optimized molecule by engineering desirable characteristics into and engineering undesirable characteristics out of the lead compound molecule. From our perspective, interest in structural molecular engineering lies with “engineering cardiotoxicity out of the molecule” to the greatest extent possible by removing or functionally disabling toxicophore(s) that lead to undesirable cardiovascular effects while maintaining the molecule’s optimal therapeutic and metabolic qualities.
Engineering out toxicophores can be considerably harder than engineering in pharmacophores, especially at the beginning of the process. While the target receptor is known, there is an enormous range of possible nontarget receptors. Some functional groups on a drug molecule may be known to comprise a toxicophore that should be avoided when designing any new drug. However, there remains the constant possibility that a functional group on a new molecule will react with a known nontarget receptor in an unexpected and undesirable manner or will interact in an undesirable manner with a previously unknown receptor. It is likely that engineering out toxicophores can be more readily achieved when the mechanism of action of a specified adverse reaction is known and is also separable from the mechanism of action of the intended response. This may be achieved by either limiting drug access to the location of the off-target receptor or by increasing the drug specificity for the on-target vs. off-target receptor (see Turner and Durham 2009).
An informative example is provided by the work of Fernández and colleagues (Fernández et al 2007). As will be seen in Chap. 10, many oncologic drugs are cardiotoxic, and considerable care is taken in the drugs’ development and also their therapeutic use. Imatinib is a small-molecule drug that is a protein–tyrosine kinase inhibitor that inhibits the bcr-bl tyrosine kinase, and it also inhibits the receptor tyrosine kinases for platelet-derived growth factor (PDGF) and stem cell factor (SCF), c-kit, and inhibits PDGF- and SCF-mediated cellular events. The drug’s specificity facilitates its treatment of chronic myeloid leukemia, where its target is the bcr-abl kinase, and also a proportion of gastrointestinal stromal tumors (GISTs), where its target is the c-kit kinase. However, it also has cardiotoxic effects traceable to its impact on the c-abl kinase. The researchers therefore engineered a “modification to imatinib that hampers Bcr-Abl inhibition; refocuses the impact on the C-Kit kinase; and promotes inhibition of an additional target, JNK, a change that is required to reinforce prevention of cardiotoxicity” (Fernández et al 2007). Imatinib was thus reengineered as an agent to treat GISTs with reduced cardiotoxicity.
2.3 A Brief Introduction to Biopharmaceuticals
The term biopharmaceutical was originally coined to define “therapeutic proteins produced by genetic engineering, rather than by extraction from normal biological sources” (Levine 2006), although the term now embraces a wider range of therapeutic interventions. The majority of approved biopharmaceuticals are proteins. Proteins are one of the topics discussed in this chapter as we provide a fundamental explanation of the biological basis of drug responses. Accordingly, additional discussion of biopharmaceutical drugs is provided in Sect. 2.12 since useful nomenclature and concepts will have been introduced by then.
2.4 Individual Variation in Responses to Drugs
One of the greatest challenges in pharmaceutical medicine is that all patients do not respond in the same manner to the same drug, a phenomenon called individual variation. When a drug is administered to different patients for whom it is appropriate as determined by the best diagnostic techniques currently available, many of these patients will safely experience a therapeutic benefit. However, there are other possible outcomes that may be experienced by relatively small numbers of patients:
A patient may not show a beneficial therapeutic response. Increasing the dose may lead to a beneficial response in some cases.
A patient may show an undesirably excessive therapeutic response (e.g., high blood pressure being reduced by an undesirable extent by an antihypertensive medication, leading to the patient becoming hypotensive instead of normotensive). A lower dose of the same drug may work well in some cases. Of interest in this scenario, the unwanted response is the result of excessive activation of the target biological structure, and hence the mechanism of action of the unwanted response is understood: this is not so easy to identify for other adverse drug reactions.
A patient may show a relatively serious adverse drug reaction that result from the drug’s interaction with an off-target receptor.
An understanding of the causes of individual variation in drug response requires knowledge of their biological underpinnings. This knowledge and pursuant understanding is being continuously enhanced by tremendous advances in molecular biology. Molecular biological aspects of drug responses are discussed later in the chapter, but first an overview of some basic biology is appropriate.
2.5 Deoxyribonucleic Acid
The acronym DNA is so well known that it is often used without reference to its full name, deoxyribonucleic acid. However, despite the ubiquity of the acronym, DNA’s nature and function may be less well known to some readers. Since genetics play an extremely influential role in both therapeutically beneficial and adverse drug responses, it is appropriate to discuss DNA at this point in the chapter.
DNA is a macromolecule. Each word in its name is descriptive of its nature. Ribose is one form of sugar, along with glucose, fructose, sucrose, and others. The prefix “deoxy-“ (the “D” in DNA) specifies a ribose that has lost one of its oxygen atoms at a specific site in the molecule. Nucleic acids (the “N” and the “A” in DNA) are a group of complex compounds comprised of carbohydrates, purines, pyrimidines, and phosphoric acid. Nucleic acids are found in all living cells.
2.5.1 Bases, Nucleotides, and Polynucleotide Strands
Each DNA macromolecule contains many copies of four bases, known as adenine (“A”), guanine (“G”), thymine (“T”), and cytosine (“C”). Each of these is a molecule in its own right, comprised of carbon, hydrogen, oxygen, and nitrogen atoms. These four bases can be meaningfully placed into two categories: pyrimidines, comprising thymine and cytosine, and purines, comprising adenine and guanine. Pyrimidines are chemical structures that are composed of one ring, and purines are chemical structures composed of two rings. Purines and pyrimidines can join together (bond) with a deoxyribose molecule that also contains a phosphoric acid group. The combination of each of the four bases (A, G, T, and C) with a deoxyribose molecule leads to the formation of four nucleotides. There are two pyrimidine nucleotides, thymine and cytosine, and two purine nucleotides, adenine and guanine. Being ring chemical structures, these nucleotides are relatively “flat,” allowing them to stack up in a compact fashion. This characteristic makes the DNA macromolecule very strong. The planar geometry of individual nucleotides, however, is in marked contrast to the eventual three-dimensional shape of DNA.
Polynucleotide strands are made up from hundreds of thousands of individual nucleotides linked together. The nucleotides in a polynucleotide strand are combined in a specific manner. The forces that hold atoms, and hence molecules, together are called bonds, and bonds occur between specific atoms in specific ways. Nucleotides in a strand are held together by bonds between the sugar component of one nucleotide and the phosphate component of the other nucleotide. Since each nucleotide has both a sugar component and a phosphate component, it can bond to one nucleotide “above” it and another nucleotide “below” it, allowing a polynucleotide chain to be formed.
2.5.2 The Double Helix and Replication
Each DNA macromolecule is comprised of two strands of nucleotides that are attached together. This molecular structure and the ensuing molecular geometry of DNA lead to its characteristic double helix nature.
Once formed, strands of DNA like to be matched with, and attached to, another strand. The process of matching and attaching is nonrandom and governed by the following rules: an adenine base can only be matched with and attached to a thymine base and a guanine base can only be matched with and attached to a cytosine base. The term “complementary bases” reflects this arrangement: each of the four nucleotide bases has a complementary base to which it becomes attached. The consequence of this is that, once the sequence of nucleotides in one strand is known, the sequence of nucleotides in the other strand is also known.
Replication is the process that starts with the two polynucleotide chains that comprise a DNA molecule splitting apart from each other. Each then becomes reattached to molecules that form a new partner, and the new partner is an exact copy of the original partner. This occurrence is governed by the complementary bases rule just described, i.e., the facts that adenine only binds with thymine and guanine only bonds with cytosine. Therefore, replication generates two precise copies of the original DNA macromolecule. These two copies can then replicate and result in four copies of the original. The exponential nature of replication means that one DNA macromolecule can produce an extremely large number of copies of itself, enough to form an entire organism.
2.6 Transmission Genetics and Molecular Genetics
Genetics, itself a relatively young science, is concerned with how traits are passed from one generation to the next. Since the advent of genomics, a more recent and hence even younger science, the term transmission genetics has come to represent what had previously been simply called genetics. The mathematics of transmission genetics were first described by Mendel in 1866 following his experiments with pea plants. It was shown that knowledge of certain phenotypic characteristics in the “parents” could be used to accurately predict phenotypic characteristics of the “offspring,” but at that time, there was no biological knowledge of the exact nature of the “units of inheritance.”
The more recent study of genes themselves is called molecular genetics. The focus of molecular genetics includes the physical and chemical structure of DNA. The information contained within our DNA codes for all of our individual characteristics. This includes eye color, blood type, susceptibility to disease, and, of particular relevance in this book, likely responses to drugs. When molecular genetic investigation expands from studying individual genes to studying the entire complement of the genome, the term genomics becomes appropriate.
2.6.1 Morgan’s Research Employing Drosophila melanogaster
Genetic experiments using Drosophila melanogaster, the fruit fly, were first conducted in 1901 at Harvard University but were taken to the next level at Columbia University by Morgan starting in 1907 (see Watson 2004). The fruit fly has marked advantages for use in transmission genetic research: they are abundant in nature, and they are easily fed and accommodated. Moreover, they are prodigious reproducers. They have a generation time of 10 days, and females produce around 300 eggs each (of which half are female). In the space of a single month (three generation times), a single fruit fly couple can lead to 150 (half of 300) times 150 times 150 flies, i.e., over 3 million flies (Watson 2004).
Since there was no current knowledge of established genetic differences in 1907, it was hard for Morgan to know where to start. As Watson (2004, p. 13) commented, “you cannot do genetics until you have isolated some distinct characteristics to track through the generations.” A search for mutant genes was therefore started. Mutant genes, now more appropriately referred to as abnormal variant genes, are variations from the normally occurring genes, which are called normal variants. Abnormal variants can lead to distinct characteristics. This search led to the discovery of some flies with white eyes (the normal color being red). This gene was therefore named white.
The work of Morgan and his colleagues laid the groundwork for the Human Genome Project. While it may initially seem a stretch to move from studying genes in fruit flies to studying genes in humans, Table 2.1 makes this very meaningful extension much more readily appreciated.
Table 2.1
Approximate genomic data for humans and other organisms of interest
Organism and date genomic data obtained | Size of genome (base pairs) | Number of genes | Percentage of genes shared with humans |
---|---|---|---|
Humans (2004) | 3 billion | 20–25,000 | (100 %) |
Chicken (2004) | 1 billion | 20–23,000 | 60 % |
Dog (2003) | 6 billion | 18,000 | 75 % |
Fruit fly (2000) | 165 million | 14,000 | 50 % |
Mouse (2002) | 2.5 billion | 30,000 | 80 % |
Rat (2004) | 2.75 billion | 22,000 | 80 % |
Twenty or so years ago, it was widely thought that the human genome may contain upward of 100,000 genes: given the complexity of human biology and behavior, it seemed reasonable (if somewhat arrogantly so) to think that humans would have a substantially larger number of genes than other species. However, following the completion of the Human Genome Project, current estimates are in the 20–25,000 range (with the most authoritative perhaps closer to the lesser figure). As can be seen in Table 2.1, the numbers of genes for several other organisms of interest are strikingly similar to the number of genes for humans. Studying the genomes of these other organisms (particularly the mouse) is advancing understanding of the human genome. Importantly, individual genes of interest in this book are preserved to a great degree across different species.
2.7 Human Genetic and Genomic Considerations
Human DNA contains approximately three billion base pairs, but our 20–25,000 genes are comprised of only a small percentage (less than 5 %) of these base pairs. That is, less than 5 % of the human genome codes for proteins: the rest is noncoding and comprises long stretches of base pairs that are often repeated. As Watson (2004, p. 197) noted, “protein-coding regions are but strings of As, Ts, Gs, and Cs embedded among all the other As, Ts, Gs, and Cs of the genome—they do not stand out in any obvious way.” To make things even more difficult when locating genes, the base pairs that comprise a gene are not arranged in an uninterrupted linear sequence: there are noncoding sequences in between the coding sequences that together form the gene. Up until the mid-1970s, it had been generally accepted that genes existed as continuous segments within a DNA molecule. This view changed radically with the discovery in 1977 that, in higher organisms with eukaryotic cells (cells that have an outer membrane and additional membranes within the cell that surround the cell’s nucleus and other intracellular organelles), an individual gene can comprise multiple DNA segments separated by chunks of noncoding DNA. This work led to researchers being awarded the 1993 Nobel Prize in Medicine.
An elegant editing process called RNA splicing (RNA is the acronym for ribonucleic acid, a molecule that shares many characteristics with DNA) removes these noncoding chunks of genetic material and connects together the relevant segments to create messenger RNA (mRNA). mRNA then ensures that amino acids are successfully made. Amino acids are joined together in various sequences to make proteins. Proteins are therefore made from the genetic instructions coded in the DNA molecule.
Noncoding sequences are called introns. A typical human gene has eight introns that lie between the coding sections, called exons, while the dystrophin gene, the largest gene found in nature, is spread across approximately 2.4 million base pairs (2.4 Mb) and is comprised of 87 exons (National Institutes of Health web site).
2.7.1 Proteins, the Proteome, and Proteomics
Genes code for proteins. That is, they contain the information that enables a sequence of amino acid residues to be joined together to form a protein. The naturally occurring amino acids are listed in Table 2.2. When a gene’s protein is manufactured, the gene is said to be expressed. Gene expression involves genes being translated into mRNA intermediates, and these intermediates then being translated into proteins, as noted in the previous section.
Table 2.2
Naturally occurring amino acids
Alanine | Glycine | Proline |
---|---|---|
Arginine | Histidine | Serine |
Asparagine | Isoleucine | Threonine |
Aspartic acid | Leucine | Tryptophan |
Cysteine | Lysine | Tyrosine |
Glutamic acid | Methionine | Valine |
Glutamine | Phenylalanine |
One aspect of gene expression that facilitates the complexity of life in higher organisms is the fact that a given gene can yield many different proteins, not just the single protein that is “classically” associated with that gene. The processes of alternative splicing and posttranslational modification make this occurrence possible. As noted in the previous section, the term splicing is used when the different exons along a section of DNA that comprise a gene are joined together once the introns separating these coding sections have been removed. Usually, exactly the same set of exons is joined together to form the gene that produces a specific protein. Alternative splicing occurs when slightly different set of exons are spliced together, resulting in the creation of a different protein.
As noted previously, only 20 amino acids are encoded genetically. Proteins (polypeptide chains) are assembled from these amino acids. The term posttranslational modification refers to biochemical changes that are made to the proteins after they have been produced. These modifications, which may be permanent or easily reversible (Glitz 2006), include glycosylation, hydroxylation, acetylation, phosphorylation, methylation, iodination, and sulfonylation. The most common protein posttranslational modification, glycosylation, involves the enzymatic attachment of sugars to the amino acid: approximately 50 % of proteins have sugars attached (Murray 2006). The number of proteins in the human proteome, the putative collection of all proteins, is therefore considerably larger than the number of genes in the genome. This phenomenon, as noted previously, is the result of “the simple although not widely appreciated fact that multiple, distinct proteins can result from one gene” (Holmes et al. 2005). Hence, the 20,000–25,000 human genes can produce many more proteins (estimates range upward of one million). Augen (2005) noted that this occurrence makes each person’s totality of metabolic pathways unique, a phenomenon that can influence drug responses.
2.7.2 The Structure of Proteins
The word protein derives from the Greek word proteios, meaning “of first importance.” Wishart (2005, p. 224) commented that “no other class of molecule exhibits the variety and irregularity in shape, size, texture, and mobility than can be found in proteins.” Proteins are described using a model comprising primary, secondary, and tertiary structures. The primary structure represents the sequence of a protein, the string of amino acids that comprise it. The individual amino acids in this chain are termed residues, and these residues are joined together by peptide bonds to form chains. This string of amino acids is shapeless and is not biologically active. Secondary structures are formed by short stretches of residues. These substructures make up sequentially proximal components of proteins, and they have shapes. A complex combination of attractive and repulsive forces between close and more distant parts of the structure affects the resultant shape of secondary structures, and predicting secondary structure from knowledge of the linear amino acid sequence alone remains a tremendous challenge. The tertiary structure addresses the overall three-dimensional structure of the protein, which represents the spatial packing of secondary structures (Ofran and Rost 2005).
Two general properties of proteins are of direct relevance to drug responses. First, once a protein is formed, it does not exist as a linear chain of its constituent amino acids: rather, it folds into very complex geometries. Second, any change to any part of the structure of a protein may have an impact on its biological activity (Thomas 2003). Proteins can comprise hundreds of amino acids, and changes in an amino acid that is a long way from the active site of the protein in terms of its function can exert a major influence on that function. The tremendous diversity in proteins, and their specificity of shape and electrical charge, may be the reason for the evolution of their role as receptors.
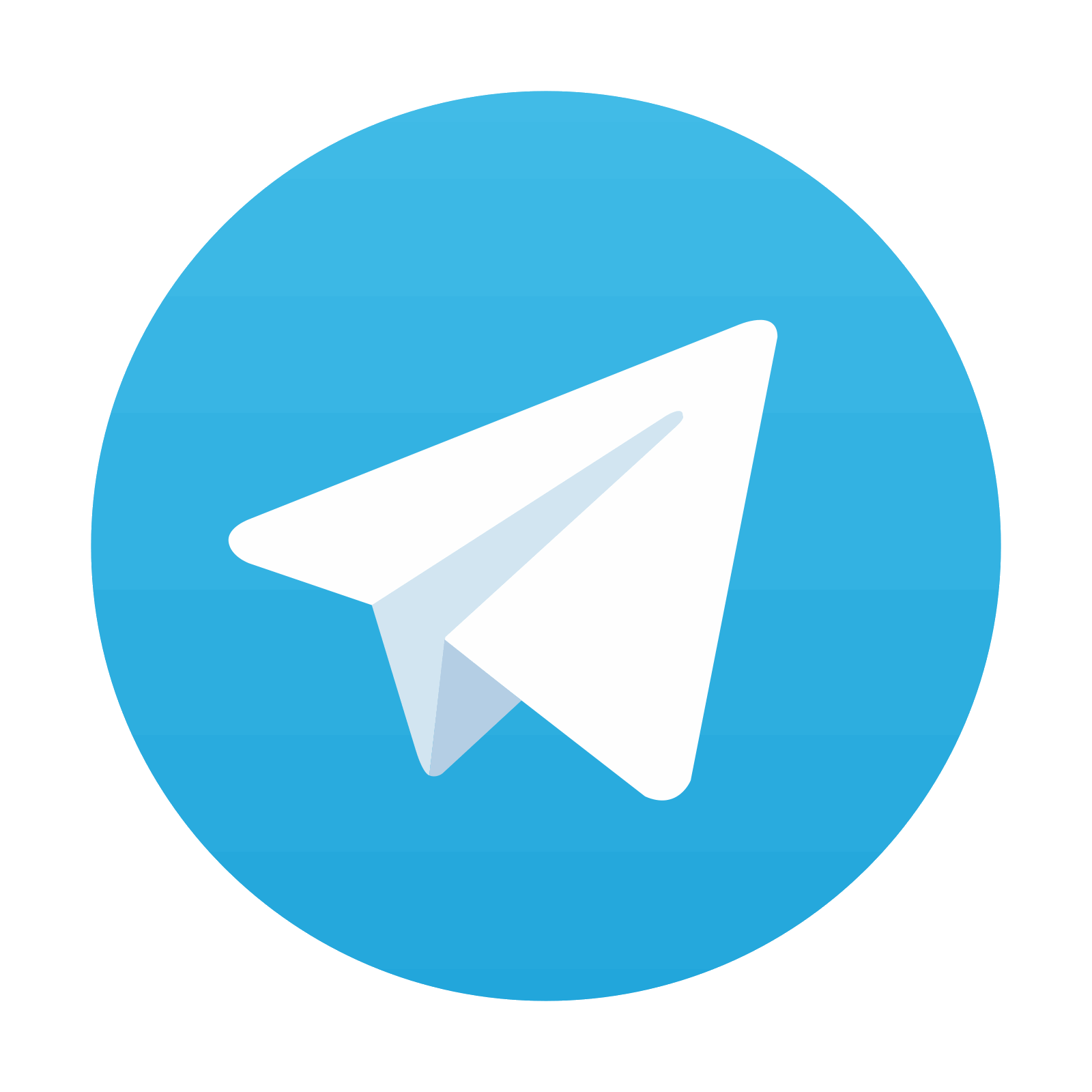
Stay updated, free articles. Join our Telegram channel
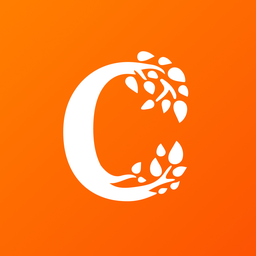
Full access? Get Clinical Tree
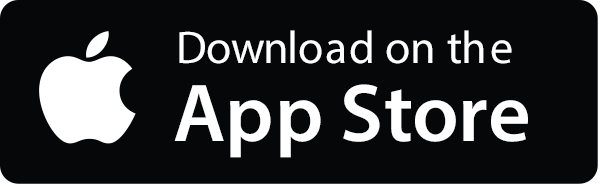
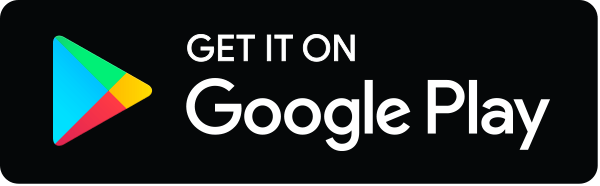