Fig. 17.1
Contemporary depiction of the blood coagulation cascade
The intrinsic pathway does not require interaction with the injured vessel wall, but rather a negatively charged surface. In the laboratory, this surface can be glass or kaolin; however, the physiologic surface is unknown [7]. Regardless, the initiating step occurs with the activation of factor XII. It is not clear what role the intrinsic pathway plays in vivo, despite understanding that deficiencies in the pathway can lead to severe bleeding disorders, such as hemophilia.
Activation of the extrinsic pathway is initiated through the release of tissue factor from the subendothelium due to injury. The released tissue factor then comes into contact with factor VII, thus beginning the cascade. There is evidence to suggest that the tissue factor-factor VII pathway is the predominant mechanism for in vivo coagulation [8]. Both routes lead to the activation of factor X, thus initiating the common pathway leading to fibrin formation and deposition resulting in a stabilized clot and secondary hemostasis.
Also within the coagulation cascade are mechanisms exerting anti-thrombotic control. Antithrombin III (ATIII) is thought to be the principal inhibitor of coagulation factors [9]. It targets most factors, notably factors IIa and Xa, and requires heparin as a cofactor to increase efficiency. Protein C is another naturally occurring anticoagulant, the effects of which stem from its ability to inactivate factors Va and VIIIa [10]. Activity of protein C requires the presence of cofactor protein S. Low levels of both protein C and S are risk factors for thrombophilia [11].
Opposing the processes of primary and secondary hemostasis in a state of equilibrium is the system of fibrinolysis. Objectively, fibrinolysis serves to break down fibrin and provide control measures on excessive or undesired thrombosis. However, excessive or unopposed fibrinolysis can lead to coagulopathy and hemorrhage. The principle component in the fibrinolysis system is the activation of plasminogen to plasmin by t-PA. Once a vessel sustains injury, t-PA, located within the endothelium, is released at the site of injury, which then initiates the pathway resulting in plasmin degradation of fibrin into fibrin split products. This process is kept localized, partly because of the specificity of t-PA for plasminogen bound to fibrin. Also, within the circulation, alpha2-antiplasmin binds circulating plasmin, thus inhibiting it and preventing systemic fibrinolysis [12].
Further inhibition of fibrinolysis is provided by mediators known as plasminogen activator inhibitors (PAIs). PAIs are contained within platelets and endothelium, and as their name implies, they bind and inhibit t-PA. Activated platelets and an injured endothelium release PAIs in high concentration. Fibrinolysis is therefore paramount. The balance between coagulation and fibrinolysis exists in a dynamic state involving many participants to achieve the desired hemostasis and prevent unwanted thrombosis. During the maintenance phase of these processes, appropriate amounts of substrate are present with normal metabolic activity. However, the stress of vascular trauma quickly alters system requirements, resulting in shifts to the dynamic state in an attempt to maintain homeostasis. Unfortunately, demand on the system often exceeds capability, which requires external forces to reestablish the delicate balance.
Impact of Vascular Trauma
Trauma to the vascular system can cause significant disruption in the flow of blood, leading to hemorrhage and thrombosis, thus initiating a complex sequence of events designed to maintain homeostasis in the setting of injury. Injuries may be penetrating or blunt trauma, with penetrating injuries being more common. Once a vessel sustains an insult, two pathophysiologic pathways are responsible for the resulting sequelae acutely. Disruption of the vessel wall can lead to active hemorrhage of whole blood, shock, and systemic inflammatory response. Damage to the layers of the vessel wall may cause cessation of blood flow, thrombosis, and distal shock. Often times, there is overlap between both pathways, creating local and systemic responses affecting coagulation [13].
Ongoing hemorrhage begins to produce signs of hemorrhagic shock once 15 % of blood volume is lost. As hemorrhage continues, signs of hypotension, tachycardia, and malperfusion intensify. Blood volume loss beyond 40 % is immediately life-threatening [14]. With the onset of hemorrhagic shock, a variety of changes begin to take place in a cascade of closely involved events.
Shock, specifically hemorrhagic shock, is a known trigger for a systemic inflammatory response, resulting in the release of inflammatory mediators secondary to injury. One of the first mediators is tumor necrosis alpha (TNF-α), and once released, it triggers the release of other mediators, such as interleukin-1 (IL-1) and IL-6. On its own, TNF-α causes vasodilation and has procoagulant activity [15]. TNF-α, IL-1, and IL-6 all invoke endothelial cells to release tissue factor (TF) and thus trigger the coagulation cascade systemically.
As expected, normal coagulation commences locally at the site of injury. However, this may not be adequate to arrest hemorrhage, and continued bleeding ensues until surgical correction. Activated clotting at sites of injury leads to consumption of platelets and coagulation factors as well as fibrin deposition. With ongoing consumption exceeding reserves, thrombocytopenia and hypofibrinogenemia occur, leading to a consumptive coagulopathy. The balance between coagulation and fibrinolysis shifts to favor clot dissolution and also explains the late observed fibrinolysis.
Bleeding trauma patients often experience hypothermia, which has known deleterious effects on coagulation. As body temperature declines, potential energy for enzymatic activity dissipates. The reactions of coagulation require enzymatic activity and are therefore slowed with decreases in body temperature. Beyond 34 °C, impairment of coagulation becomes significant [16]. The level of derangement is not evident on routine laboratory tests as the samples are warmed to 37 °C prior to testing. Platelet function is also impacted by hypothermia. In addition to reduction in platelet enzymatic function, production of thromboxane A2 is reduced, which results in vasodilation and reduced platelet aggregation, leading to microvascular bleeding [17].
Mechanisms of DIC
Disseminated intravascular coagulation is a syndrome describing systemic observances due to derangements in activated coagulation and fibrinolysis and occurs because of some underlying etiology [18]. Trauma is a known initiator of DIC, which is found in 50–70 % of trauma patients [19]. DIC can be variable, from mild to life-threatening, and has identifiable stages indicating constant progression. Clinically, manifestations include thrombosis, coagulopathy, hemorrhage, and multiorgan system failure. Once initiated, coagulation derangements can become severe, and goal of therapy is to treat the underlying cause and restore the normal balance between coagulation and fibrinolysis.
The precipitating event in DIC is tissue factor (TF) activation of the coagulation cascade leading to fibrin deposition. Mediators, such interleukin-1 (IL-1), IL-6, and tumor necrosis factor alpha (TNF-α), play a key role in the release of TF from the subendothelium [20]. The subsequent activation of the extrinsic pathway via factor VIIa ultimately leads to generation of factor Xa and extensive thrombin formation and fibrin deposition [21]. In addition, the resulting endothelial damage results in platelet activation and aggregation, causing platelet consumption.
Normal opposing mechanisms to thrombosis are also impaired in DIC. Levels of antithrombin III are found to be low in DIC. Normal levels of protein C and S are also decreased [20]. Furthermore, fibrinolysis activity is depressed secondary to increased levels of plasminogen activator inhibitor (PAI) [22]. This systemic coagulation=exaggerated activation leads to consumption of substrate for coagulation and defines phase I of DIC [23]. Microthrombi also form within organ system vascular beds, causing thrombosis and organ ischemia, which lead to organ dysfunction.
As phase I continues, consumption ultimately exceeds available reserves, and the system of coagulation begins to decompensate, defining phase II of DIC. At this point, laboratory evaluation demonstrates increased prothrombin time (PT) and partial thromboplastin time (PTT). However, thrombin time (TT) may still be normal because of the remaining normal levels of fibrinogen. Further ongoing consumption eventually exhausts the system completely, resulting in phase III of DIC [23]. Once reaching this point, laboratory clotting times are markedly increased, platelet counts have dropped, and fibrinogen levels are now also low, as are levels of other coagulation factors. With extreme systemic coagulation insufficiency, generalized hemorrhage is an associated clinical finding with phase III DIC.
< div class='tao-gold-member'>
Only gold members can continue reading. Log In or Register a > to continue
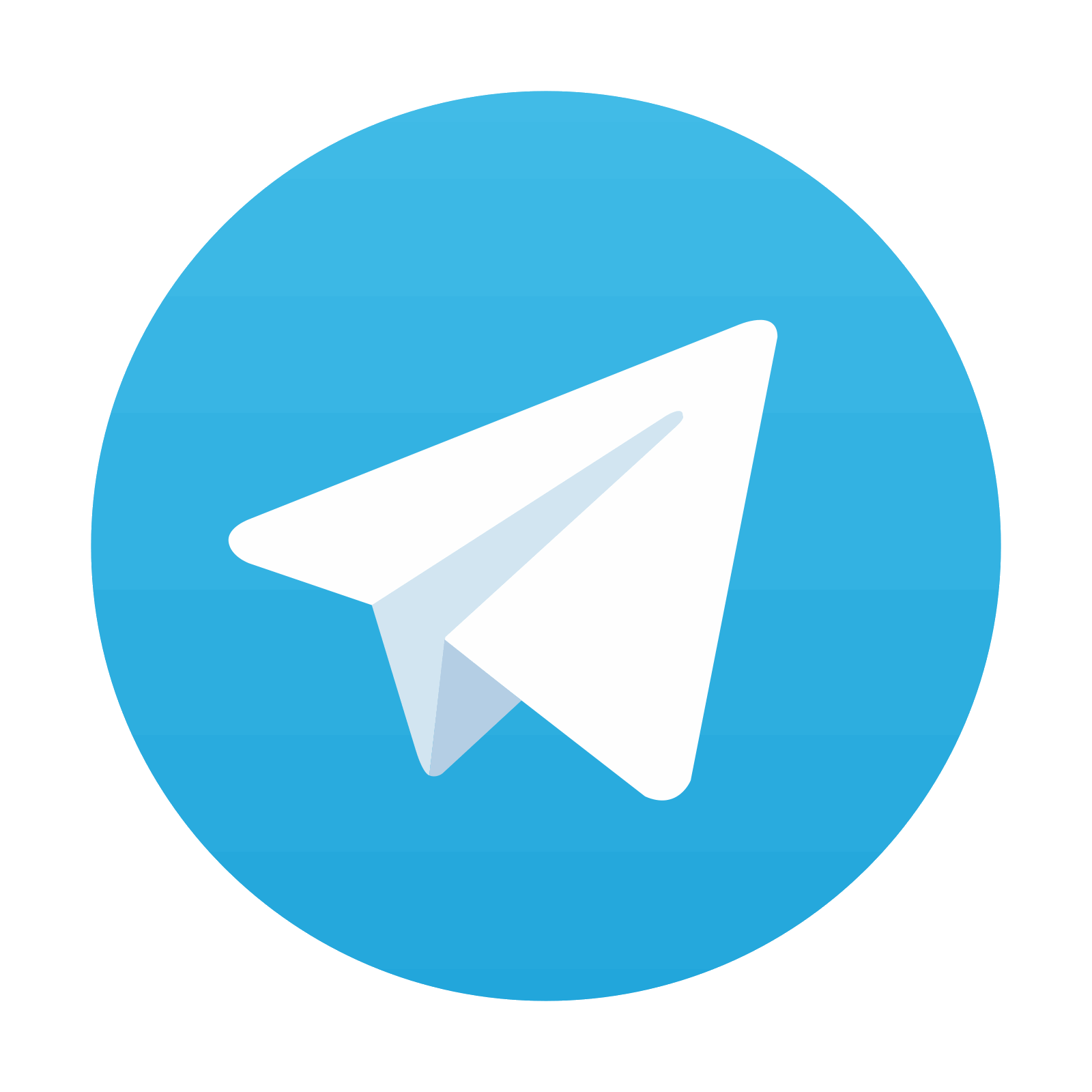
Stay updated, free articles. Join our Telegram channel
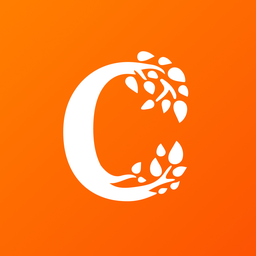
Full access? Get Clinical Tree
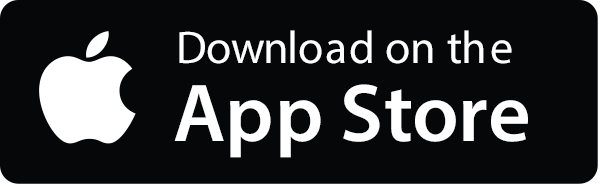
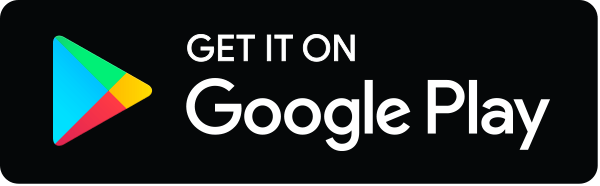