Surgical therapy for aortic arch disease involves partial or complete replacement of the arch with great vessel reimplantation during a period of time when native cerebral blood flow is temporarily interrupted. In its original description, this interval of suspended cerebral perfusion required the cessation of blood flow to the entire body and was labeled hypothermic circulatory arrest (HCA). HCA employs the use of systemic hypothermia to protect the brain and visceral organs during the ischemic period, and provides a bloodless operative field to facilitate arch reconstruction. Since the initial report of the use of HCA for aortic arch replacement, cerebral protection, and circulation management techniques have evolved to enable safe, reproducible arch reconstruction with excellent neurologic outcomes.1
HCA traces its beginnings to the work of Bigelow who demonstrated a role for hypothermia in cardiac surgery in a canine model.2 John Lewis used systemic hypothermia at 28°C combined with venous inflow occlusion to perform the first successful atrial septal defect repair. He subsequently described the use of profound systemic hypothermia (9°C) with one hour of circulatory arrest without any evidence of central nervous system injury to treat an adult with ovarian carcinoma.3,4 Systemic hypothermia was temporarily abandoned with the arrival of the cardiopulmonary bypass machine, but was rediscovered and used in isolated reports of arch replacement in the 1960s.5,6 However, it was the translational research of Dr. Randall Griepp, who applied lessons learned from his canine laboratory models to successfully repair aortic arch aneurysms using HCA, that ushered in the modern era of aortic arch surgery.1
For the purposes of clarity throughout this chapter, the use of HCA without adjunctive cerebral perfusion will be termed as “HCA alone”. The classification system in Table 15-1 will be used to describe varying degrees of hypothermia used for HCA Table 15-1. Deep hypothermic circulatory arrest (DHCA) and moderate hypothermic circulatory arrest (MHCA) are the two levels of HCA that will be primarily focused upon throughout the subsequent text.
Comprehension of HCA mandates a fundamental understanding of cerebral metabolism and blood flow. The metabolic rate and energy requirements of the brain are approximately 7.5 times the metabolism of non-nervous system tissues. The brain depends upon the aerobic process of glycolysis for energy and is estimated to have only a 2-minute supply of glucose stored as glycogen in neurons at any time. The brain is intolerant of ischemia and in that setting quickly resorts to anaerobic metabolism for energy production. Once adenosine triphosphate (ATP) stores are depleted, basic cellular ionic pumps fail and calcium accumulates in the intracellular space. This leads to irreversible neuronal injury.7
In order to support the high cerebral metabolic rate, the body provides approximately 60 mg of glucose and 3.5 mL of oxygen per 100 mg of brain tissue per minute. This translates to a cerebral blood flow of 750 to 900 mL/min or 15% of resting cardiac output under normothermic conditions.7 The autoregulatory capacity of the cerebral microcirculation ensures constant flow rates in normotensive individuals within a mean arterial blood pressure range of 60 to 140 mm Hg. This level of perfusion maintains a ratio of cerebral blood flow to metabolism of 20:1. However, several physiologic variables including temperature, pH, and hematocrit can modulate cerebral blood flow.8
Since oxygen is vital to brain metabolism, measurement of the cerebral metabolic rate for oxygen (CMRO2) provides important comparative information regarding the metabolic state of the brain. Hypothermia has been shown to exponentially reduce cerebral metabolism. Systemic hypothermia at 20°C reduces the CMRO2 to 76% of its baseline, and deeper hypothermia to 15°C reduces CMRO2 to 84% of baseline values (Table 15-2).9 In contrast to the CMRO2, hypothermia reduces cerebral blood flow in a linear fashion. As the core body temperature decreases to 22°C, the cerebral microcirculation loses its ability to autoregulate and cerebral blood flow becomes dependent upon mean arterial pressure.10 Loss of autoregulation translates into an uncoupling of cerebral blood flow and metabolism. This produces excess or “luxury” perfusion of the brain, and the ratio of cerebral blood flow to metabolism increases to a ratio of 75:1. The significance of luxury perfusion is unclear. Potential beneficial effects of luxury perfusion include a quicker, more homogenous cooling of the brain; however, potential detrimental effects include the development of brain edema and exposure to an increased embolic load.8
Temperature (°C) | Cerebral metabolic rate (% of base line) | Safe duration of HCA (min) |
---|---|---|
37 | 100 | 5 |
30 | 56 (52-60) | 9 (8-10) |
25 | 37 (33-42) | 14 (12-15) |
20 | 24 (21-29) | 21 (17-24) |
15 | 16 (13-20) | 31 (25-38) |
10 | 11 (8-4) | 45 (36-62) |
The most clinically accessible method of assessing cerebral metabolism is the measurement of cerebral electrical activity, which can be monitored by electroencephalography (EEG). Systemic cooling diminishes cerebral electrical activity and electrocerebral or EEG silence indicates maximal cerebral metabolic suppression. Many aortic centers use complete EEG silence during the cooling period as a reliable and safe measure of adequate cerebral suppression, indicating that it is safe to initiate HCA.
As stated previously, cerebral blood flow is impacted by temperature and the acid-base status of the blood. Two different strategies are used during HCA to manage pH. Alpha-stat pH management maintains normal acidemia and blood gases in 37°C (normothermic) blood. This optimizes cellular enzyme activity and preserves cerebral autoregulation. In alpha-stat management, the pH is allowed to change as the blood temperature decreases, and the blood becomes more alkaline and hypocapneic. Such conditions cause a shift of the oxyhemoglobin dissociation curve to the left, which increases the affinity of hemoglobin for oxygen. The net effect is that in deep hypothermia, oxygen bound to hemoglobin is less available to be released to the tissues, and the fraction of oxygen dissolved in blood represents the major source of oxygen to the tissues.
The alternative strategy is pH-stat management, which maintains a normal pH at hypothermia by adding CO2 to the blood during cooling. Supplementary CO2 causes cerebral vasodilatation and eliminates the autoregulatory capacity of the cerebral microvasculature. This results in an increase in cerebral blood flow and an uncoupling of cerebral blood flow from cerebral metabolism. Due to the supplementary CO2, at deep hypothermia, the oxyhemoglobin dissociation curve is shifted to the right, which decreases the affinity of hemoglobin to oxygen and increases the delivery of oxygen to the tissues. Under pH-stat management, the blood becomes acidotic and hypercapneic when the blood temperature is rewarmed to 37°C.
Studies comparing the two strategies have been conducted in both large animals and humans. The majority of human studies contain small numbers of patients who underwent either adult cardiac or congenital heart procedures that did not utilize circulatory arrest. The adult studies are composed of patients undergoing coronary artery bypass grafting under moderate-to-mild levels of hypothermia (26-32°C). Conclusions from the adult studies indicate superior outcomes with alpha-stat management. The animal and pediatric studies contain data conducted under deep hypothermia with either low flow cardiopulmonary bypass or circulatory arrest. Outcomes in these studies were superior with pH-stat management.11
The mechanisms of intraoperative brain injury differ between adults and children. Adults are prone to suffer embolic injury secondary to risk factors for vasculopathy (eg, hypertension, atherosclerosis, diabetes mellitus). Children undergoing repair of complex congenital heart disease are more susceptible to global ischemic injury from prolonged periods of low flow or circulatory arrest. Therefore, alpha-stat management may be more optimal in adults, where the preservation of cerebral autoregulation enables the microvasculature to adjust to areas of stenoses and any disproportionate distribution of flow. Conversely, children may benefit from the global cerebral vasodilatation associated with pH-stat management to augment blood flow during prolonged low flow states.11-13
It is well recognized that there are two different types of brain injury observed in patients treated with HCA: (1) permanent neurologic dysfunction (PND) and (2) temporary neurologic dysfunction (TND). PND, commonly referred to as stroke, manifests clinically as a focal neurologic deficit secondary to an embolism of particulate matter or air/gas bubbles causing vascular occlusion resulting in an ischemic cerebral infarct. Risk factors for PND in patients undergoing HCA include atheromatous disease involving both the ascending aortic and the site of arterial cannulation for cardiopulmonary bypass.14-16 The axillary artery has been shown to provide the lowest risk of stroke in patients undergoing HCA operations.16 Other neuroprotective strategies to reduce the risk of embolic stroke include the use of epiaortic ultrasound to guide the site of arterial cannulation and aortic cross clamping, and the addition of antegrade or retrograde cerebral perfusion (RCP).
TND is a reversible, diffuse subtle injury which is attributed to inadequate cerebral protection.17 Patients with TND can experience confusion, agitation, delirium, prolonged obtundation, orparkinsonism without localizing signs in the immediate postoperative period. Brain imaging in this clinical setting with computed tomography or magnetic resonance imaging is negative. TND is the clinical manifestation of a global cerebral ischemic injury that results in necrotic and/or apoptotic neuronal cell death.
Necrotic cell death following HCA occurs due to a failure to meet minimum cerebral cellular metabolic and oxygen requirements. In this instance, cellular stores of ATP are exhausted and the cell converts to anaerobic metabolism. The lack of ATP also causes a failure of the Na+/K+ pump which has a multitude of consequences including cellular swelling, depolarization of the cell membrane, opening of voltage sensitive Ca2+ channels and a massive influx of calcium into the cytoplasm. Accumulation of intracellular calcium activates proteases and lipases that disrupt the cellular membrane and results in cell lysis and death. Histologically, cellular necrosis is characterized by pyknotic nuclei, swollen eosinophilic cytoplasm, and the presence of an inflammatory reaction. Risk factors for neuronal cell necrosis following HCA include inadequate levels of hypothermia, the presence of electrocerebral activity at the time of circulatory arrest, and prolonged duration (>40 minutes) of HCA without cerebral perfusion.18-20
The second type of cell death observed in patients after HCA with inadequate cerebral protection is apoptosis. Apototic cell death is an energy-dependent process that occurs despite adequate stores of cellular energy. It represents a sub-lethal ischemic injury that results in the activation of specific genes, receptors and enzymes that break down the cell in a programmed manner.21 Morphologically it is characterized by nuclear karyorrhexis, and margination of chromatin in the nucleus with minimal cytoplasmic or inflammatory changes. Apoptosis is a highly sophisticated and complex process that has not yet been fully characterized, and a detailed description is beyond the scope of this chapter. Fundamentally, it is a carefully regulated process that leads to the generation of caspases which initiate a proteolytic cascade in which one caspase activates other caspases and leads to rapid cell death.22 Multiple animal models of DHCA have demonstrated apoptotic cell death beginning within hours of reperfusion and continuing as long as 72 hours after DHCA.23,24
Ischemia and hypoxia can initiate cell death through a third mechanism related to excessive neuronal stimulation and hyperactivity. Once the brain converts to anaerobic metabolism, lactate is produced. The inability of the neuronal cells to utilize lactate as an energy source, combined with the lack of blood flow during HCA, results in the accumulation of lactate and a drop in intracellular pH. The development of intracellular acidosis has a multitude of effects including the release of the potent excitatory neurotransmitters glutamate and aspartate, and failure of the neurotransport pumps. This results in the accumulation of glutamate in the intercellular space. Glutamate in increasing concentrations becomes neurotoxic and mediates the opening of calcium channels. The end result is an influx of calcium into the cytoplasm, which initiates a lethal biochemical cascade resulting in neuronal cell death. Excitotoxicity is the term applied to this type of neuronal cell death induced by excitatory amino acids. Excitotoxicity plays an important role in both necrotic and apoptotic ischemic neuronal cell death.18 Metabolic rates differ throughout the different regions of the brain, and foci with high metabolic rates are more sensitive to ischemia. This concept has been termed “selective vulnerability” and, applies to neurons in the hippocampus, basal ganglia and cerebellum.25,26 Patients with TND due to inadequate cerebral protection following HCA can often display poor cognition, altered short-term memory, and fine motor deficits. Neuropsychological tests in patients undergoing HCA have demonstrated memory and fine motor deficits consistent with subtle brain injury in patients following periods of DHCA > 25 minutes.27 This type of subtle brain injury is likely due to necrotic or apoptotic neuronal cell death in the hippocampus, basal ganglia, and cerebellum. The impact of TND is not clinically insignificant and correlates with long-term memory and motor deficits following prolonged periods of DHCA.28
After the initiation of cardiopulmonary bypass, systemic hypothermia is achieved by cooling the blood passing through a heat exchanger connected to the perfusion circuit. A temperature gradient (arterial inflow to venous return) that does not exceed 10°C is maintained during the cooling period to prevent the formation of gaseous emboli. The duration of the cooling period required to equilibrate blood and tissue temperatures is highly variable and is dependent upon blood flow, temperature gradient (between perfusate and organs), and tissue-specific coefficients of temperature exchange. Patient-specific variables that impact cooling include occlusive vascular disease and body mass index.
There is no consensus regarding cooling strategies for HCA. Intraoperative monitoring of time, temperature, jugular venous bulb oxygen saturation, and electroencephalographic activity can assist in the evaluation of cerebral metabolic suppression during the cooling period. Temperature is monitored in many different sites including the inflow and outflow lines of the perfusion circuit, and by probes inserted into the bladder, rectum, nasopharynx, and esophagus. If the cerebral protection strategy is HCA alone or in conjunction with RCP, then the cooling strategy should be based upon esophageal or nasopharyngeal temperatures, as these sites have been shown to closely approximate brain temperature.29 However, if antegrade cerebral perfusion is used, bladder or rectal temperatures are more important, as visceral organ protection becomes the primary goal of hypothermia.
Generally accepted parameters that ensure sufficient cerebral metabolic suppression include cooling for a minimum of 30 minutes, a goal nasopharyngeal temperature of 18°C, a jugular venous saturation > 95%, and electrocerebral silence.30-32 However, relying on such parameters alone as a measure of adequate metabolic suppression may not be completely adequate. In a study of 109 patients undergoing HCA, >50% of patients had not achieved electrocerebral silence at a nasopharyngeal temperature of 18°C, and approximately 25% of patients have not achieved electrocerebral silence after 30 minutes of cooling.33 This emphasizes the need to thoroughly evaluate all available monitoring parameters prior to initiating HCA. If HCA alone is employed, topical cooling by packing the head in ice is advocated in order to minimize upward drift of intracranial temperatures during the ischemic period.31
Cerebral reperfusion and rewarming following the period of HCA is a critical phase that can exacerbate neuronal injury if not conducted properly. Following HCA, reinitiating brain reperfusion with a short period of low-pressure cold blood flow prior to rewarming is recommended. In contrast to immediate rewarming, cold reperfusion has been associated with improved cerebral perfusion, a reduction in intracranial pressure, and a decrease in cerebral edema. This strategy attenuates the adverse effects of the impaired cerebral vascular autoregulation that occurs following DHCA, and results in a reduction in brain injury.34,35 Experimental data have also suggested that reperfusion should also be carried out with a higher hematocrit and normoglycemia. A lower hematocrit in the reperfusate (≈20%) has been shown to increase the degree of histopathologic brain injury compared to a higher (30%) hematocrit.36 In addition to an increased oxygen carrying capacity, a higher hematocrit is thought to be beneficial due to an increase in the buffer, redox and free-radical scavenging capacity. Hyperglycemia during reperfusion has been correlated with worsening intracellular acidosis, which prevents the return of normal cellular metabolism.37
Oxygen delivery to the brain is particularly important during the reperfusion period as there is a mismatch between cerebral metabolism and blood flow. Cerebral vascular resistance is increased for up to 8 hours following the period of HCA. This results in a reduction in cerebral blood flow and an abnormally high extraction rate of oxygen and glucose from the blood in order to sustain cerebral metabolism.38 It is during this period that secondary brain injury can occur with episodes of hypotension, hypoxemia, and anemia.31 A gradient < 10°C must be maintained to prevent the formation of gas emboli, and the temperature of the perfusate should not exceed 36°C. Cerebral hyperthermia results in worse neurobehavioral outcomes and increased neuronal cell injury compared to mild hypothermia following DHCA.39 The mechanisms behind this injury are poorly understood; however, it is likely multi-factorial and related to increases in the permeability of the blood-brain barrier and cellular metabolism in the setting of hyperthermia. The risk of this delayed neurologic injury is significant, and many high volume aortic centers advocate a period of permissive hypothermia in the intensive care unit following DHCA in an attempt to reduce this risk.18,40
“DHCA alone” or DHCA without adjunctive cerebral perfusion represents the simplest, most convenient form of cerebral protection. It does not require complex perfusion strategies or neuromonitoring, and is the cerebral protection strategy of choice for surgeons who infrequently perform aortic arch reconstructions. The main concern surrounding the use of DHCA alone is the duration of the circulatory arrest period.
In an attempt to provide data regarding the safe interval of circulatory arrest at different temperatures, the Mount Sinai group measured arterial and venous blood gases at various time and temperature points in 37 adults undergoing HCA. This led to the calculation of the CMRO2 consumption at various temperatures. Using CMRO2, the Q10 (a well-described physiologic variable describing the temperature-dependent decrease in cerebral metabolism), and the assumption that cerebral blood flow can be safely interrupted for 5 minutes at 37°C, a calculated safe duration of HCA was determined for a range of temperatures. Figure 15-1 demonstrates the relationship between CMRO2, temperature, and the calculated safe duration of HCA.9,18
FIGURE 15-1
Limits of ‘safe’ duration of circulatory arrest. Q10 for the adult brain calculated from direct measurement of cerebral metabolic rate for oxygen (CMRO2) in 37 adult patients undergoing thoracic aortic operations with DHCA. The temperature-related reduction in the metabolic rate and the calculated ‘safe’ periods of arrest are shown. (Data from McCullough JN, Zhang N, Reich DL, et al: Cerebral metabolic suppression during hypothermic circulatory arrest in humans, Ann Thorac Surg. 1999 Jun;67(6):1895-1899.)
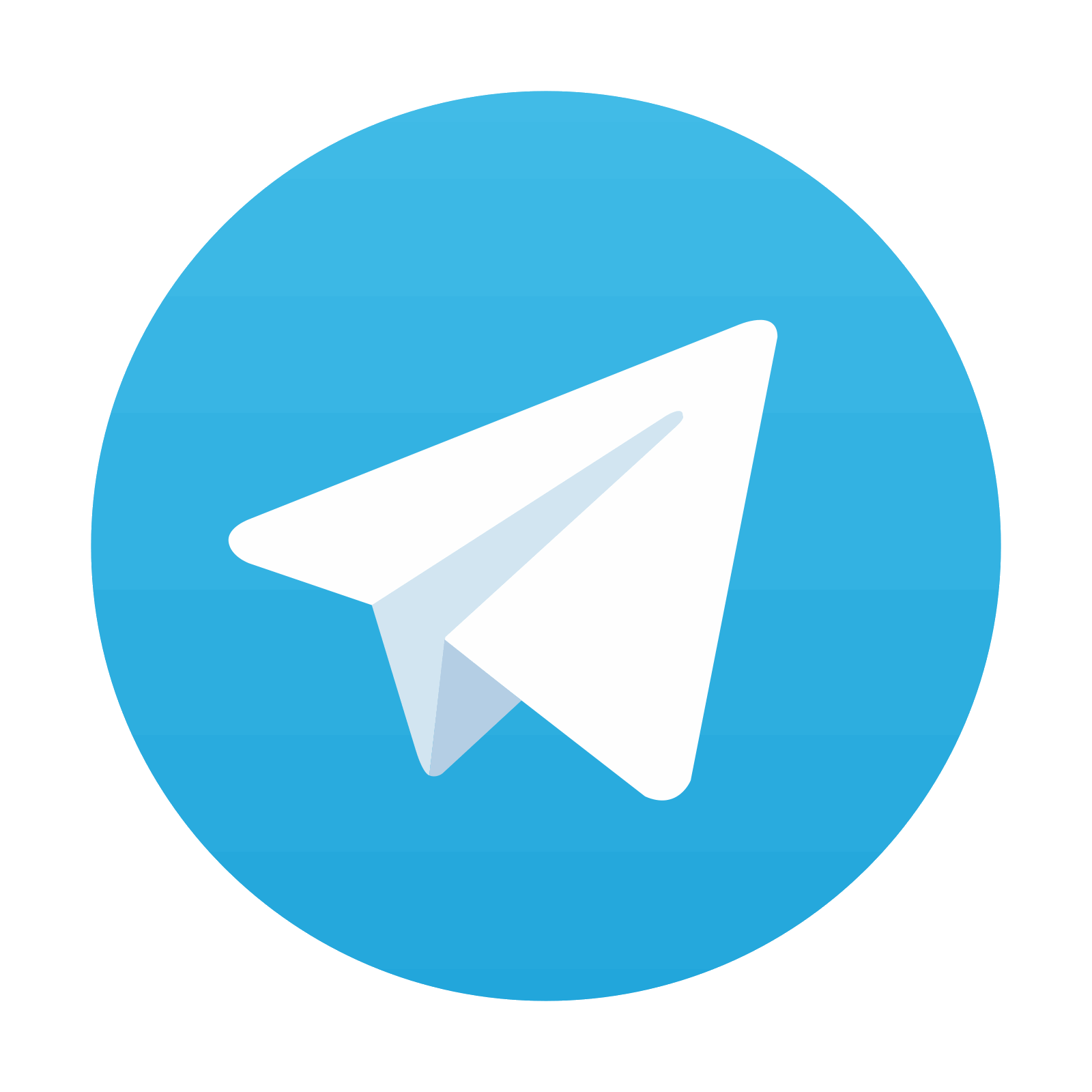
Stay updated, free articles. Join our Telegram channel
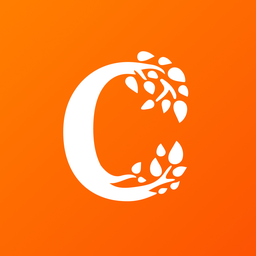
Full access? Get Clinical Tree
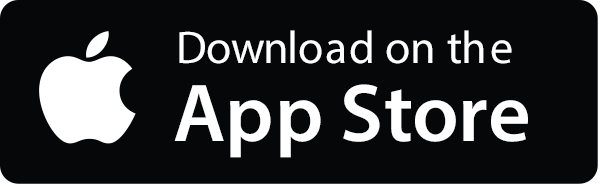
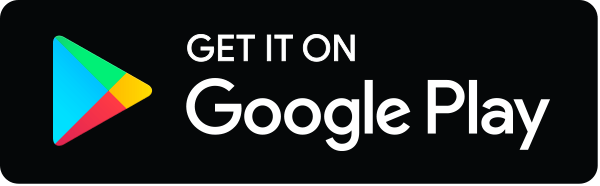
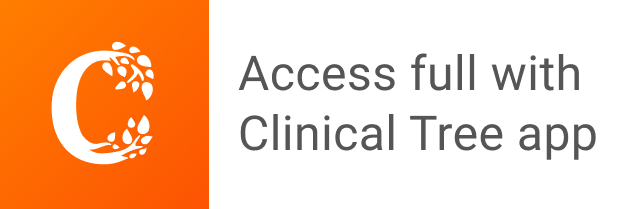