Fig. 45.1
Immature dendritic cells mature into antigen-presenting cells (APCs) within recipients’ lymph nodes and spleen. APCs present donor alloantigens on their class II MHC molecules. CD4 helper T cells recognize alloantigens with the use of their surface immunoglobulins and MHC class II molecules. Activated subgroups of helper T cells have distinct cytokine profiles to promote rejection or tolerance. APC antigen-presenting cell, MHC major histocompatibility complex, TCR T-cell receptor, IL Interleukin, IFN-γ interferon-γ, Treg regulatory T cell, TGF-β transforming growth factor β
Initially, donor’s antigen-presenting cells (APC) recognize donor-derived antigens located on the cells of the allograft. APCs migrate from the allograft to the recipient lymphoid tissue and present donor major histocompatibility complex class II and B7 complex to the recipient’s T-cells for direct allorecognition [32, 33]. Allorecognition is mediated by the recognition of foreign MHC molecules on the surface of donor cells by recipient dendritic cells [37]. T cell-dendritic cell interaction requires local cytokine expression. Intracellular activation of calcineurin leads to production of IL-2. In fact, blockade of IL-2R has been used as an effective therapeutic strategy promoting allograft survival [38].
Active APCs produce IL-12. IL-12 initiates CD4+Th1 activation and stimulates the development of activated CD8+ cells and interferon (IFN)-γ production both in vivo and in vitro [39, 40]. IFN-γ activates macrophages, upregulates the expression of MHC class II antigen, and promotes allograft rejection [41]. In their enlightening study, Saiura and colleagues have revealed a distinct upregulating effect on the genes that are involved in the inflammatory process after HT, while failing to document any conclusive findings on the regulation of chemokine ligand 9 and chemokine ligand 10 in mice. They also reported upregulation of allograft inflammatory factor-1 throughout the investigated time points (days 3, 5, and 7) following HT. Most importantly, samples taken from patients with acute graft rejection displayed the central role of IFN-γ in inducing several chemokines. Macrophage inflammatory protein 1 alpha expression is shown to increase during rejection in both wild-type and IFN-γ-deficient mice, while the rejection process in IFN-γ-deficient mice did not show increased expressions of CXCL9 and CXCL10 [42].
IL-12 and -23 share the p40 subunit and are crucial for the development of T helper (Th) 1 and Th17 cell responses in acute graft rejection [43]. Th17 cells, a recently identified CD4+ effector T cell subset, produce proinflammatory IL-17 in both mice and humans [44]. Furthermore, Xie and colleagues [43] demonstrated effective inhibition of Th1 and Th17 cell responses after treatment with an anti-IL-12/23p40 antibody in vivo mouse model of acute cardiac allograft rejection. Recently, Wang and colleagues [45] demonstrated that the levels of Th1, Th17, and FoxP3+ CD4+ T cells and their specific transcription factors increased in patients with acute rejection after HT and were associated with rejection grades.
T-helper 1 (Th1)-type cytokines (e.g., IL-2, IFN-γ, IL-12, TNF-α) are closely linked in the elimination of intracellular infections and mediating allograft rejection [46]. TNF-α -308 SNP is associated with acute cellular rejection following HT [47]. In contrast, the Th2 cytokine profile (IL-4, -5, and -10) is associated with allograft tolerance. For example, IL-10 promoter at positions −1,082, −819, and −592 correlates with increased IL-10 production [48]. Allograft rejection is characterized by CXCR3 carrying T cells and, more importantly, release of Th1-related cytokines and expression of CXC chemokines that are inducible by IFN-γ, for example, monokine induced by interferon gamma Mig/CXCL9, interferon-inducible T-cell alpha chemoattractant I-TAC/CXCL11 and interferon and IFN-γ -inducible protein-10 IP-10/CXCL10 [49]. In brief, allograft rejection is related to Th1 cell trafficking caused by CXCR3-binding chemokines. CXCL9 and CXCL10 are documented to be involved in the development of acute allograft rejection [50]. While CXCL10 mediates the congregation of leukocytes, it also acts as an initiator of the alloimmune response against the antigen, taking a critical part in the initiation of an inflammatory loop, which fuels the immune response in graft rejection [51]. Interestingly, high levels of pre-transplant CXCL10 in serum have been proposed to serve as a biomarker for predicting the risk of acute allograft rejection in the first 3 months after the transplantation [52]. Recently, Sathya and colleagues [53] showed a significant correlation with post-transplant circulating endothelial progenitor cell (EPC) function, namely colony-forming units and rejection episodes.
MicroRNAs (miRs) have recently emerged non-coding RNAs that can regulate gene expression at the posttranscriptional level and key regulators for the immune system [54–56]. MiRs have been demonstrated to play important roles in regulating dendritic cell function, B cell and T cell-mediated rejections, and Treg activities [57]. A recent study showed a significant correlation with MHC class I-related gene A (MICA) upregulation and histological evidence of severe rejection after HT in 44 patients [58].
Diagnosis of Rejection
Endomyocardial biopsy (EMB) is currently the gold standard method for detection and confirmation of the allograft rejection. Several methods have been proposed for an accurate diagnosis, yet none so far has proven to be adequate or specific enough to replace the status quo [59]. The necessity to replace endomyocardial biopsy is to avoid an invasive method and to provide the clinician with a tool with fast and accurate results. In current practice, major adjunct methods are used for monitoring rejection, such as electrocardiography, electrophysiology, radio-isotopic techniques, cyto-immunologic monitoring, and magnetic resonance imaging [60, 61]. Among the proposed biochemical markers are urinary polyamines [61], neopterins [62], prolactin [63], beta-2-microglobulins [64], and brain natriuretic peptide (BNP) [65], none of which had fulfilled the need for sensitivity.
New high-throughput analyses that utilize protein or gene expression analysis techniques, cell-mediated immune assays, and fluorescence-activated cell sorter analysis of cellular subpopulations have the potential to provide the clinicians with more accurate markers of allograft rejection. Advancements in microarray technologies and bioinformatics allow researchers to determine specific proteins, genes, metabolites and pathways that are involved in normal and dysfunctional processes [66]. These major methodological developments create the possibility for discovery of new markers, while also presenting novel targets for therapy. Human biopsy materials obtained from ailing tissue are being used to investigate the etiology of diseases; a good example would be cardiac biopsies used for tetralogy of Fallot [67] and atrial fibrillation [68]. New biomarkers can be developed by utilizing such methodologies and provide stepping stones for new therapy options. DNA microarray experiments involving animal models have been performed to understand the Brown Norway to Lewis heterotopic heart transplant model [69]. A recent study has documented a correlation between endomyocardial biopsy material and whole blood by using microarray analysis. A new biomarker panel from whole blood samples is proposed for detection of rejection, which was claimed to have as efficient results as biopsy microarray [70].
Cardiac Allograft Vasculopathy
Despite the major improvements that have been achieved in the prevention and treatment of acute transplant rejection, long-term survival following HT has not improved. Cardiac allograft vasculopathy (CAV) is the major long-term limitation of HT survivors [71, 72]. Despite a 2–4 % recent decrease in the cumulative incidence of CAV, the prevalence of CAV still remains high. CAV is detectable by angiography in 8 % of the patients within the first year, 20 % at 3 years, 32 % at 5 years, and 45 % at 8 years following HT [21]. Briefly, CAV is the leading cause of late morbidity and mortality in HT patients, accounting for 30 % mortality in the first 5 years [21]. The pathogenesis of CAV is not fully elucidated. Indeed, CAV is a complex, multifactorial phenomenon. Immunologic (indirect allorecognition, upregulation of cytokines and cytokine-related adhesion molecules, focal inflammation, and vasculitis) and nonimmunologic factors (repetitive vascular injury) may play critical roles in the process [32, 73–75].
EMB and coronary angiography provide effective but invasive monitoring methods for CAV in cardiac transplantation recipients. Histologically CAV is characterized by a concentric and diffuse intimal thickening composed of T cells, macrophages, and modified smooth muscle cells beneath an activated endothelium in epicardial and intramural coronary arteries along their entire lengths [76–83]. The disease begins as concentric fibrous intimal thickening caused by myofibroblast proliferation and fibrosis mainly in the proximal region of epicardial arteries [80]. Following the first year of HT, intermediate lesions with intimal lipid-filled cell accumulation can be detected. In long-term survivors, fibrous and fibrofatty intimal lesions often diffusely involve large and small epicardial and intramural arteries [80–83]. Late increase in TNF-α and IFN-γ in endomyocardial biopsies precedes the development of CAV [84]. van Loosdregt and colleagues demonstrated abundant CD4+ T cells that express HLA DR in transplanted hearts with CAV [85]. IL-17 has been shown to be critical in TGFbeta-driven allograft fibrosis [86]. Recently, elevated levels of circulating smooth muscle progenitor cells (SPCs), but not EPCs, and elevated plasma CXCL12 concentrations were found in CAV patients in contrast to patients without CAV [87]. Furthermore, the presence of coronary inflammatory plaque as assessed by virtual histology intravascular ultrasound (VH-IVUS) was associated with early recurrent rejection and with subsequent progression of CAV [88]. Metabolic syndrome is associated with endothelial dysfunction and increases the risk of CAV [89]. New-generation immunosuppressive drugs and immunomodulatory medication may have beneficial effects on coronary microvasculature following heart transplantation [90].
Conflicts of Interest
There is no undisclosed ethical problem or conflict of interest related to the submitted manuscript.
References
1.
Carrel A, Guthrie CC. The transplantation of veins and organs. Am Med. 1905;10:1101–2.
2.
Lower RR, Shumway NE. Studies on orthotopic homotransplantation of the canine heart. Surg Forum. 1960;11:18–9.PubMed
3.
Shumway NE, Lower RR. Topical cardiac hypothermia for extended periods of anoxic arrest. Surg Forum. 1960;10:563–6.PubMed
4.
5.
9.
10.
Lower RR, Stofer RC, Hurley EJ, Shumway NE. Complete homograft replacement of the heart and both lungs. Surgery. 1961;50:842–5.PubMed
11.
Barnard CN. The operation. A human cardiac transplant: an interim report of a successful operation performed at Groote Schuur Hospital, Cape Town. S Afr Med J. 1967;41(48):1271–4.PubMed
13.
Barnard MS, van Heerden J, Hope A, O’Donovan TG, Barnard CN. Total body perfusion for cardiac transplantation. S Afr Med J. 1969;43(3):64–7.PubMed
14.
Brink JG, Hassoulas J. The first human heart transplant and further advances in cardiac transplantation at Groote Schuur Hospital and the University of Cape Town – with reference to: the operation. A human cardiac transplant: an interim report of a successful operation performed at Groote Schuur Hospital, Cape Town. Cardiovasc J Afr. 2009;20(1):31–5.PubMed
15.
16.
17.
18.
19.
Cooley DA, Frazier OH, Kahan BD. Cardiac transplantation with the use of cyclosporin a for immunologic suppression. Tex Heart Inst J. 1982;9(3):247–51.PubMed
20.
Pennock JL, Oyer PE, Reitz BA, Jamieson SW, Bieber CP, Wallwork J, et al. Cardiac transplantation in perspective for the future. Survival, complications, rehabilitation, and cost. J Thorac Cardiovasc Surg. 1982;83(2):168–77.PubMed
21.
Stehlik J, Edwards LB, Kucheryavaya AY, Benden C, Christie JD, Dobbels F, et al. The Registry of the International Society for Heart and Lung Transplantation: Twenty-eighth Adult Heart Transplant Report – 2011. J Heart Lung Transplant. 2011;30(10):1078–94. doi:10.1016/j.healun.2011.08.003.PubMedCrossRef
22.
Stehlik J, Edwards LB, Kucheryavaya AY, Aurora P, Christie JD, Kirk R, et al. The Registry of the International Society for Heart and Lung Transplantation: twenty-seventh official adult heart transplant report–2010. J Heart Lung Transplant. 2010;29(10):1089–103. doi:10.1016/j.healun.2010.08.007.PubMedCrossRef
< div class='tao-gold-member'>
Only gold members can continue reading. Log In or Register a > to continue
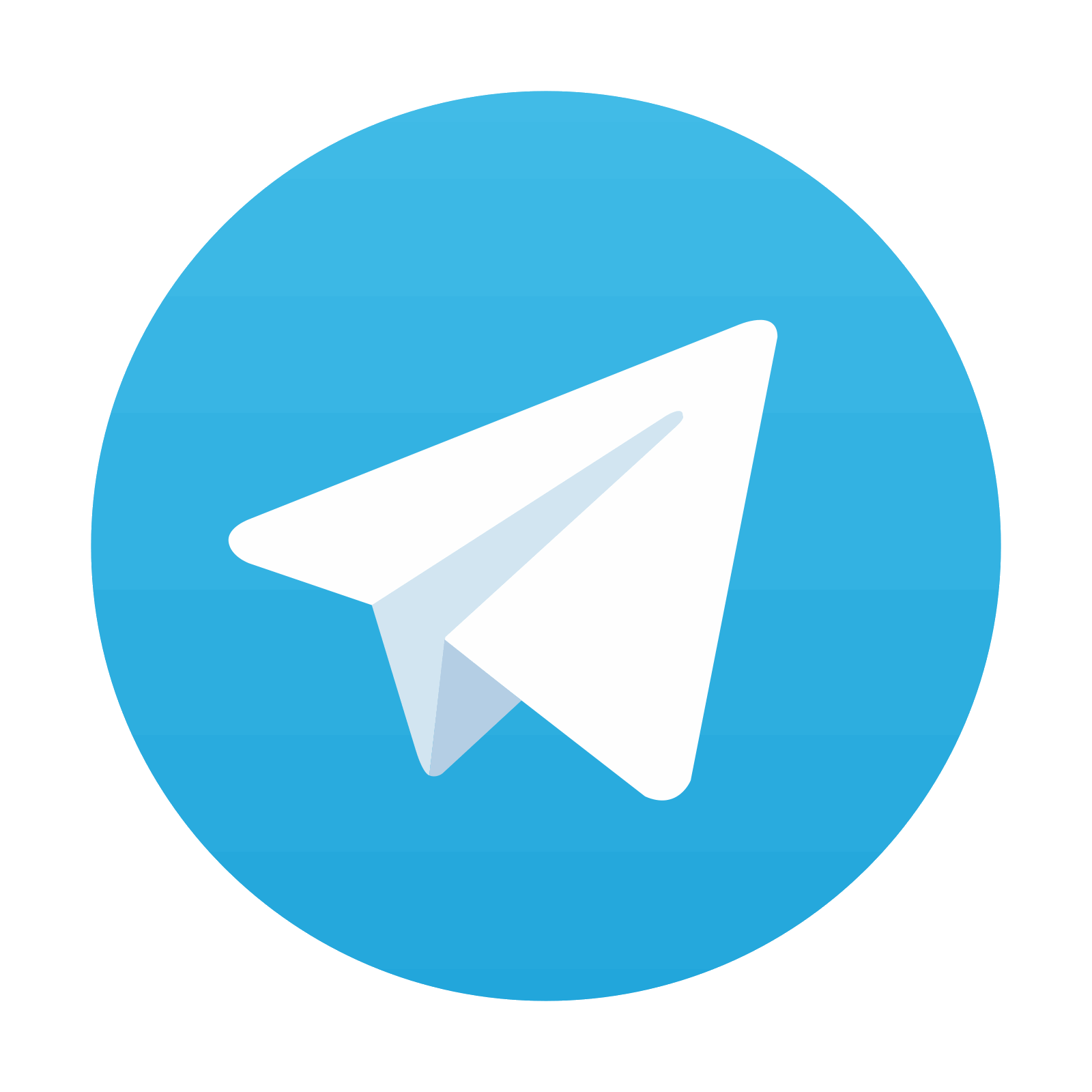
Stay updated, free articles. Join our Telegram channel
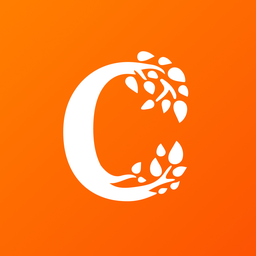
Full access? Get Clinical Tree
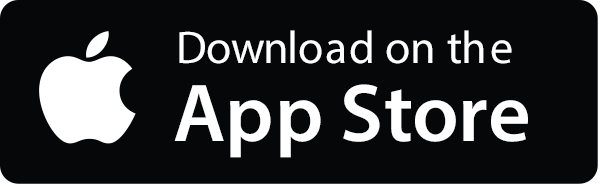
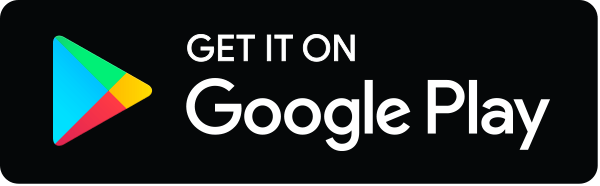