With the developments in ultrasound transducer technology and both hardware and software computing, real-time volumetric imaging has become widely available, accompanied by various methods of assessing three-dimensional (3D) myocardial strain, often referred to as 3D speckle-tracking echocardiographic methods. Indeed, these methods should provide cardiologists with a better view of regional myocardial mechanics, which might be important for diagnosis, prognosis, and therapy. However, currently available 3D speckle-tracking echocardiographic methods are based on different algorithms, which introduce substantial differences between them and make them not interchangeable with each other. Therefore, it is critical that each 3D speckle-tracking echocardiographic method is validated individually before being introduced into clinical practice. In this review, the authors discuss differences and similarities of the currently available 3D strain estimation approaches and provide an overview of the current status of their validation.
Ultrasonic myocardial deformation imaging offers the possibility of quantifying regional cardiac deformation noninvasively. It is widely used for the detection of myocardial ischemia and has been proposed as a tool to detect heart disease at its preclinical stage, to differentiate among various hypertrophy etiologies, and to monitor therapy.
Traditionally, cardiac motion and deformation analysis was limited to a visual inspection of the image sequence, in which each myocardial segment was assigned a wall motion score. However, this methodology depended considerably on the expertise of the interpreter and showed relatively low interobserver agreement.
For this purpose, myocardial strain (i.e., the relative lengthening or shortening of the myocardial segment expressed as a percentage of its initial length) and strain rate (i.e., the rate of this lengthening or shortening) imaging was introduced. For further details, the reader is referred to previous literature. At present, the assessment of one-dimensional strain with Doppler tissue imaging (DTI) is a well-established technique to regionally quantify myocardial function. This technique requires high frame rates, which may be difficult to obtain in dilated ventricles. However, it can be overcome by reducing the imaging sector width. Therefore, this should not be considered a true limitation of the technique. On the other hand, just as with other Doppler techniques, this technique is angle dependent, as only the strain component along the ultrasound beam can be measured. Although it allows the measurement of longitudinal strain (LS) in all left ventricular (LV) segments, radial strain (RS) and circumferential strain (CS) can only be measured in a limited number of LV segments.
Non-Doppler-based, two-dimensional (2D) strain estimation methods can partly overcome these limitations. Various echocardiographic techniques have been proposed, which use raw radiofrequency signals or apply block-matching techniques, often termed speckle-tracking (ST) techniques, on B-mode images. Several of these methods based on 2D ST have already been commercialized. However, six acquisitions of different parasternal and apical LV views are still required to obtain all strain components in all LV segments. This can be an issue in certain experimental and clinical situations, particularly when heart rate or LV loading conditions change rapidly.
With developments in ultrasound transducer technology and both hardware and software computing, systems capable of acquiring real-time volumetric LV data are now widely available. Most of the systems use electrocardiographic (ECG) gating to construct three-dimensional (3D) data from smaller subvolumes acquired at subsequent cardiac cycles (i.e., stitching). As such, reasonable spatial and temporal resolution of 3D data sets can be achieved. The ability to estimate true 3D myocardial motion and deformation using various 3D ST echocardiography (STE) approaches may provide cardiologists with a better view of regional myocardial mechanics, which may be important for diagnosis, prognosis, and therapy. These 3D approaches can measure all strain components in all LV segments from a single acquisition. Furthermore, they are angle independent, do not suffer from strain estimation errors associated with out-of-plane motion, and may in theory allow more precise calculations of LV twist and assessment of shear strain components.
However, tracking in three dimensions naturally poses some challenges. The increased field of view of volumetric images comes at the cost of both spatial and temporal resolution of the data set. In other words, the current volumetric data sets show a coarser speckle pattern and a higher speckle decorrelation between subsequent volumes. The latter effect becomes even more pronounced in data sets acquired at high heart rates (e.g., during stress echocardiography). Moreover, because of the amount of data available in volumetric data sets, the computational load is also higher compared with 2D data sets. These aspects pose a challenging environment for myocardial motion and deformation imaging.
Given the potential of 3D STE techniques and a multitude of publications that have recently appeared in medical journals, we felt that a comprehensive overview that would make this area more accessible to the reader was lacking. In this review, we thus discuss differences and similarities among the currently available 3D STE approaches and provide an overview of their first validation and clinical application studies.
Three-Dimensional Strain Estimation Approaches
General Work Flow
All currently available techniques follow the same subsequent steps to estimate 3D strain. This is illustrated in Figures 1 to 4 .




In the initial step, key cardiac events are indicated: end-systole and end-diastole. This can be done automatically (e.g., on the basis of the electrocardiogram) or manually (e.g., by visually inspecting the cardiac cycle and indicating the frames with the smallest and largest volumes, respectively; Figure 1 ). Second, the region of interest for strain estimation is defined. Depending on the software, the endocardial border, sometimes in combination with the epicardial border, is delineated manually, semiautomatically, or automatically. Typically, this involves contouring the end-diastolic frame, although some software packages may require repeating the process for the end-systolic frame as well ( Figure 2 ). Next, the left ventricle is subdivided into segments for segmental strain analysis, usually ranging from 16 to 18 segments depending on the segmentation model used. An anatomic landmark (e.g., the right ventricular insertion point) is used to indicate the orientation of the segments ( Figure 3 ). Finally, the 3D LV region of interest is tracked throughout the cardiac cycle, and the deformation curves are estimated ( Figure 4 ). The main differences between the currently available 3D STE algorithms are found in this final step. Their underlying principles are briefly discussed in the next sections.
Block Matching
The most common 3D STE approach is based on block matching, which can be considered a direct extension of 2D ST to three dimensions. This technique is based on the assumption of a stable local speckle pattern between subsequent volumes. Local tissue motion can then be extracted from the displacement of those speckle patterns from one frame to another ( Figure 5 ). Because the motion estimates are performed independently from one another, they are usually noisy. An a posteriori regularization step (e.g., by spatially smoothing the initial motion estimates) is therefore often performed. Note that this approach also works on volumetric data acquired through ECG gating, given that no stitching artifacts appear in the volumetric data (otherwise, the assumption of a stable speckle pattern between subsequent frames is violated).

It is a popular approach because of its conceptual simplicity and high computational speed. Several commercial implementations of this method are available.
Elastic Registration
Elastic or nonrigid image registration methods use image-warping techniques to estimate cardiac motion between subsequent volumes. In this method, one image is deformed in multiple steps to look as similar as possible to the next image in the image sequence. Once both images match optimally, interframe motion is known ( Figure 6 ). As opposed to the block-matching approaches, the motion of the whole myocardium is thus estimated simultaneously. However, not all warping solutions are feasible or desired. Therefore, additional conditions are imposed during the motion estimation process (e.g., by enforcing the tissue motion to be smooth in space and/or time). For more details, the reader is referred to previously published articles discussing the technical aspects of elastic image registration. Currently, this method has been used mostly in academic circles and will be made commercially available.

Model-Based Approach
Models incorporating a priori knowledge can also be used to guide the 3D myocardial motion and strain estimation process. Different sources may be used as input to this model; for example, a biomechanical model can describe the deformation of myocardium, incorporating the presence of myocardial fibers, or a statistical model can describe the typical shape of the left ventricle, its typical appearance (i.e., the local grayscale properties of the myocardium), or even its typical motion.
Using a statistical model for motion estimation consists of two stages ( Figure 7 ). The first step involves building a large annotated database containing manually segmented volumetric data sets of healthy and pathologic hearts. This database is then used to learn the normal and pathologic geometry and appearance or motion of a ventricle offline. In the second step, myocardial motion in a new data set can be estimated online by combining and comparing the image data with the database. The expertise of the clinical experts delineating the ventricles is thus captured and used as a priori knowledge.
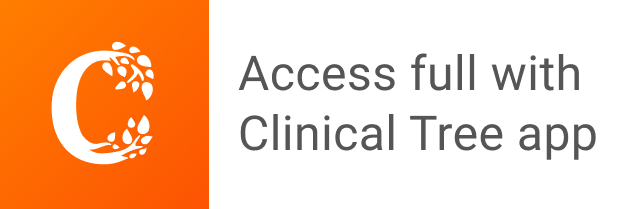