Attention ASE Members:
ASE has gone green! Visit www.aseuniversity.org to earn free continuing medical education credit through an online activity related to this article. Certificates are available for immediate access upon successful completion of the activity. Nonmembers will need to join the ASE to access this great member benefit!
Table of Contents
Abstract 277
Abbreviations 278
1. Terms and Definitions: Basic Parameters of Myocardial Function 278
2. Techniques Used to Assess Local Cardiac Chamber Wall Dynamics
2.1. Doppler Tissue Imaging (DTI) 278
2.2. Two-Dimensional (2D) Speckle-Tracking Echocardiography (STE) 283
2.3. Three-Dimensional (3D) STE 286
2.4. Integrated Backscatter (IBS) Analysis 288
3. Physiologic Measurements of Left Ventricular Function
3.1. LV Architecture and Vectors of Myocardial Deformation 290
3.2. Clinical Use of LV Displacement, Velocity, Strain, and SR 292
3.3. LV Rotation 293
3.4. LV Dyssynchrony 295
3.5. LV Diastolic Function 298
3.6. Myocardial Ischemia 298
3.7. Fibrosis and Viability 300
4. Physiologic Measurements of Right Ventricular and Left and Right Atrial Function
4.1. Right Ventricle 303
4.2. Left Atrium 308
4.3. Right Atrium 311
5. Conclusions 311
Table of Contents
Abstract 277
Abbreviations 278
1. Terms and Definitions: Basic Parameters of Myocardial Function 278
2. Techniques Used to Assess Local Cardiac Chamber Wall Dynamics
2.1. Doppler Tissue Imaging (DTI) 278
2.2. Two-Dimensional (2D) Speckle-Tracking Echocardiography (STE) 283
2.3. Three-Dimensional (3D) STE 286
2.4. Integrated Backscatter (IBS) Analysis 288
3. Physiologic Measurements of Left Ventricular Function
3.1. LV Architecture and Vectors of Myocardial Deformation 290
3.2. Clinical Use of LV Displacement, Velocity, Strain, and SR 292
3.3. LV Rotation 293
3.4. LV Dyssynchrony 295
3.5. LV Diastolic Function 298
3.6. Myocardial Ischemia 298
3.7. Fibrosis and Viability 300
4. Physiologic Measurements of Right Ventricular and Left and Right Atrial Function
4.1. Right Ventricle 303
4.2. Left Atrium 308
4.3. Right Atrium 311
5. Conclusions 311
Abstract
Echocardiographic imaging is ideally suited for the evaluation of cardiac mechanics because of its intrinsically dynamic nature. Because for decades, echocardiography has been the only imaging modality that allows dynamic imaging of the heart, it is only natural that new, increasingly automated techniques for sophisticated analysis of cardiac mechanics have been driven by researchers and manufacturers of ultrasound imaging equipment. Several such techniques have emerged over the past decades to address the issue of reader’s experience and intermeasurement variability in interpretation. Some were widely embraced by echocardiographers around the world and became part of the clinical routine, whereas others remained limited to research and exploration of new clinical applications. Two such techniques have dominated the research arena of echocardiography: (1) Doppler-based tissue velocity measurements, frequently referred to as tissue Doppler or myocardial Doppler, and (2) speckle tracking on the basis of displacement measurements. Both types of measurements lend themselves to the derivation of multiple parameters of myocardial function. The goal of this document is to focus on the currently available techniques that allow quantitative assessment of myocardial function via image-based analysis of local myocardial dynamics, including Doppler tissue imaging and speckle-tracking echocardiography, as well as integrated backscatter analysis. This document describes the current and potential clinical applications of these techniques and their strengths and weaknesses, briefly surveys a selection of the relevant published literature while highlighting normal and abnormal findings in the context of different cardiovascular pathologies, and summarizes the unresolved issues, future research priorities, and recommended indications for clinical use.
1. Terms and Definitions: Basic Parameters of Myocardial Function
Displacement , d , is a parameter that defines the distance that a certain feature, such as a speckle or cardiac structure, has moved between two consecutive frames. Displacement is measured in centimeters.
Velocity , v , reflects displacement per unit of time, that is, how fast the location of a feature changes, and is measured in centimeters per second.
Strain , ϵ, describes myocardial deformation, that is, the fractional change in the length of a myocardial segment. Strain is unitless and is usually expressed as a percentage. Strain can have positive or negative values, which reflect lengthening or shortening, respectively. In its simplest one-dimensional manifestation, a 10-cm string stretched to 12 cm would have 20% positive strain.
Strain rate , SR, is the rate of change in strain and is usually expressed as 1/sec or sec −1 .
Displacement and velocity are vectors ; that is, in addition to magnitude, they have direction . Thus, one can examine their different spatial components along the x, y, and z directions, or alternatively along the anatomic coordinates of the cardiac chambers, longitudinal, radial, and circumferential components, which are especially relevant for the characterization of myocardial mechanics.
Similar logic applies to strain and SR, which provide local information on myocardial deformation. The important advantage of strain and SR over displacement is that they reflect regional function independently of translational motion. Nevertheless, deformation imaging cannot distinguish active from passive deformation. The term “principal strain” describes the local magnitude and direction of the shortening or lengthening of the myocardium. The term “global strain” or, more precisely, “global longitudinal strain” or “global circumferential strain” usually refers to the average longitudinal or circumferential component of strain in the entire myocardium, which can be approximated by the averaged segmental strain components in individual myocardial wall segments. Strain values can be expressed for each segment (“segmental strain”), as an average value for all segments (“global strain” mentioned above), or for each of the theoretical vascular distribution areas (“territorial strain”).
The term left ventricular (LV) rotation refers to myocardial rotation around the long axis of the left ventricle. It is rotational displacement and is expressed in degrees. Normally, the base and apex of the ventricle rotate in opposite directions. The absolute apex-to-base difference in LV rotation is referred to as the net LV twist angle (also expressed in degrees). The term torsion refers to the base-to-apex gradient in the rotation angle along the long axis of the left ventricle, expressed in degrees per centimeter.
2. Techniques Used to Assess Local Wall Dynamics
2.1. Doppler Tissue Imaging (DTI)
Since the early attempts to implement the concept of tracking tissue motion using Doppler ultrasound and the subsequent development of DTI over the past two decades, this imaging technique has been used by multiple investigators to advance the understanding of cardiac pathophysiology and test a variety of potential new diagnostic techniques, as evidenced by a large body of literature. Although many of these techniques remained limited to the research arena, some have won widespread recognition and become mainstream tools in the arsenal of clinical echocardiography.
Although continuous-wave Doppler analyzes the frequency shift of the returning echoes compared with the original frequency of the ultrasound beam ( Figure 1 A), both pulsed-wave and color Doppler imaging use the phase shift between consecutive echoes for the velocity calculation. In pulsed-wave Doppler mode, ultrasound wavelets are emitted repeatedly at a certain repetition frequency along a single scan line, and the returning echoes are sampled at a preset time after each pulse is sent, allowing the determination of the distance between the target and the transducer. The amplitude of the sampled echoes over time is then converted into a velocity spectrum using a fast Fourier transform ( Figure 1 B). In color Doppler mode, the echo of the entire scan line is received and divided into several range gates. To determine the phase shift between pulses in all range gates, an auto-correlation algorithm is used to convert the phase shifts into velocity values, which are displayed in a color overlay of the image ( Figure 1 C). Although pulsed-wave Doppler has the advantage of offering a direct curve display during the examination, only color Doppler allows postprocessing, including tracking of the sample volume and calculating derived parameters (e.g., displacement or SR).
Tissue Doppler velocity estimation is based on the same principles as pulsed-wave and color Doppler echocardiography for blood flow. To distinguish between signals originating from moving tissue and blood flow, a so-called wall filter is used, which is a high-pass filter used to image blood velocities or a low-pass filter used to display tissue velocities. While the intensity of the signals generated by the myocardium is higher than that generated by blood, blood velocity usually exceeds that of the myocardium.
DTI Acquisition
Spectral Doppler acquisition requires setting the sample volume size and position so that it remains within the region of interest inside the myocardium throughout the cardiac cycle. Scale and baseline should be adjusted in a way that the signal fills most of the display. Sweep speed must be adjusted according to the application for measuring slopes and time intervals: high sweep speed for measuring slopes in a few beats and low sweep speed for measuring peak values in several beats. Some imaging systems enable retrospective adjustments of sweep speed in stored data without a loss of data quality. Gain should be set to a value that produces an almost black background with just some weak noise speckles, to ensure that no important information is lost. On the other hand, caution should be taken to avoid excessive gain, as this causes spectral broadening and may cause overestimation of peak velocity. Although cardiac motion is three dimensional and complex, Doppler methods can measure only a single component of the regional velocity vector along the scan line. Care should therefore be taken to ensure that the ultrasound beam is aligned with the direction of the motion to be interrogated ( Figure 2 A). The angle of incidence should not exceed 15°, thereby keeping the velocity underestimation to <4%. Only certain motion directions can be investigated with Doppler techniques ( Figure 2 B). In LV apical views, velocity samples are usually obtained at the annulus and at the basal end of the basal and mid levels and less frequently in the apical segments of the different walls.
DTI Acquisition
Color Doppler requires a high frame rate, preferably >100 frames/sec, and ideally ≥140 frames/sec. This can be achieved by reducing depth and sector width (ideally both grayscale and Doppler sectors) and by choosing settings that favor temporal over spatial resolution. Usually, the image is optimized in the grayscale display before switching to the color mode and acquiring images. Care should be taken to avoid reverberation artifacts by changing interrogation angle and transducer position, because such artifacts may affect SR estimations over a wide area ( Figure 3 ). The velocity scale should be set to a range that just avoids aliasing in any region of the myocardium. Slowly scrolling through the image loop before storing allows recognition of possible aliasing. As with spectral Doppler, the motion direction to be interrogated should be aligned with the ultrasound beam. If needed, separate acquisitions should be made for each wall from slightly different transducer positions. Data should be acquired over at least three beats, that is, covering at least four QRS complexes and stored in a raw data format. Older imaging systems that store only color values in an image should not be used unless postprocessing is not planned. Acquisition of blood flow Doppler spectra of the inlet and outlet valves of the interrogated ventricle provides useful information for timing of opening and closing of the valves, and thus for hemodynamic timing of measurements obtained from the time curves of various parameters. For sufficient temporal matching, all acquisitions should have similar heart rate and show the same electrocardiographic lead.
DTI Image Analysis
Spectral Doppler data cannot be further processed. Peak velocities, slopes, and time intervals are measured directly on the spectral display. Figure 4 shows normal velocity time curves obtained from the LV basal septum and lateral wall.
DTI Image Analysis
Color Doppler data can be displayed and postprocessed in different ways. Various function parameters can be derived from a predefined region of interest within the same color Doppler data set, including velocity, displacement, SR, and strain ( Figure 5 ). Two display concepts are used: color coding with or without a straight or curved M-mode and reconstructed curves of regional function. Color-coded data are best interpreted in still frames, particularly in M-mode displays. This way, there is easy visual access to the regional and temporal distribution of a particular parameter within the wall. Curve reconstructions are subsequently possible from any point within a stored data set ( Figure 5 ). This allows displays of the exact time course of regional velocities and other parameters. An advantage of color Doppler processing over pulsed-wave Doppler is that with postprocessing, the sample volume can be adjusted to track the motion of the myocardium, thus staying in the same region throughout the entire cardiac cycle. Another advantage is that a sampling of the different myocardial regions is possible in the same time.
Color Doppler Measurements of Myocardial Function
Because Doppler imaging generates velocity information, velocity, v , at any location and any time can be obtained directly from the color Doppler data.
Displacement, d , can be obtained by calculating the temporal integral of the tissue velocity, v :
Because of the nature of Doppler imaging, d describes only the motion component of the tissue in the sample volume toward or away from the transducer, while components perpendicular to the beam remain unknown. Thus, the motion curve of the mitral annulus derived from color Doppler data should have the same shape and magnitude as the M-mode tracing of the mitral annulus obtained in the same location.
SR is the temporal derivative of strain. Analytically, it is identical to the spatial gradient of tissue velocity and can therefore be obtained from the color Doppler data, as the difference between velocities measured in two samples 1 and 2 divided by the distance r between the two samples :
Strain can be calculated as the temporal integral of SR with appropriate mathematical adjustments :
Time curves can be generated from color Doppler data for each spatial component (i.e., longitudinal, radial, and circumferential) of each of the above four parameters of cardiac function ( Figure 6 ).
The time course of a spectral Doppler velocity curve is similar to a color Doppler–derived one. However, absolute values differ because the spectral curve is usually measured at the outer edge of the spectrum, whereas color Doppler data approximate the mean velocity of a region, so that reported pulsed Doppler peak velocity is typically 20% to 30% higher than that measured by color Doppler. Accordingly, it is recommended that the modal velocity (the brightest or darkest line in the spectral display, depending on display) be used for pulsed Doppler measurements.
Potential Pitfalls of DTI
Tissue Doppler velocities may be influenced by global heart motion (translation, torsion, and rotation), by movement of adjacent structures, and by blood flow. These effects cannot be completely eliminated but may be minimized with the use of a smaller sample size (which may, however, result in noisier curves) and with careful tracking of the segment. To minimize the effects of respiratory variation, the patient should be asked to suspend breathing for several heartbeats.
The tissue Doppler signal can be optimized by making the width of the imaging beam as narrow as possible. Although temporal resolution is excellent with M-mode and spectral tissue Doppler, it is not as good with color tissue Doppler because of the lower frame rate.
The apical views are best for measuring the majority of LV, right ventricular (RV), and atrial segments in a parallel-to-motion fashion, although there may be some areas of deficient spatial resolution, for instance, near the apex, because of the prevalence of artifact and problems with proximal resolution. In the parasternal long-axis and short-axis views, tissue Doppler assessment is impossible in many segments (e.g., in the inferior interventricular septum and in the lateral wall) because the ultrasound beam cannot be aligned parallel to the direction of wall motion. Modified views should be used whenever necessary to achieve the optimal imaging angle.
Displacement and deformation of the myocardium are cyclic processes with no defined beginning or end. Therefore, the position of the baseline (zero line) is arbitrary. Most analysis packages define zero automatically as the value at the beginning of the QRS complex (red arrows in Figures 5 B and 5 D) and report the actual position or length change relative to that value. Although useful for multiple applications, this approach may not work under certain circumstances (bundle branch blocks, wrong QRS detection on the electrocardiogram, atrial fibrillation, etc.). Care must be taken in such cases to clearly define and mark the beginning time point of deformation analysis or denote (perhaps by manual editing) the baseline (zero line) so that comparable references are used during repeated studies.
Furthermore, the integration used to calculate displacement and strain often results in an erroneous baseline shift. Most software programs automatically apply a linear correction, which is often referred to as drift compensation ( Figure 7 ).
Strengths and Weaknesses of DTI
The major strength of DTI is that it is readily available and allows objective quantitative evaluation of local myocardial dynamics. Over the past decade, this ability triggered extensive research in a variety of disease states that affect myocardial function, either globally or regionally, as reflected by the large body of literature involving this methodology. It is well established that peak tissue velocities are sufficiently reproducible, which is crucial for serial evaluations. Also, spectral pulsed DTI has the advantage of online measurements of velocities and time intervals with excellent temporal resolution, which is essential for the assessment of ischemia (see section 3.6) and diastolic function (see section 3.5). The major weakness of DTI is its angle dependency, as any Doppler-based methodology can by definition only measure velocities along the ultrasound beam, while velocity components perpendicular to the beam remain undetected. In addition, color Doppler–derived strain and SR are noisy, and as a result, training and experience are needed for proper interpretation and recognition of artifacts.
2.2. Two-Dimensional (2D) Speckle-Tracking Echocardiography (STE)
STE is a relatively new, largely angle-independent technique used for the evaluation of myocardial function. The speckles seen in grayscale B-mode images are the result of constructive and destructive interference of ultrasound backscattered from structures smaller than the ultrasound wavelength. With this technology, random noise is filtered out, while keeping small temporally stable and unique myocardial features, referred to as speckles. Blocks or kernels of speckles can be tracked from frame to frame (simultaneously in multiple regions within an image plane) using block matching, and provide local displacement information, from which parameters of myocardial function such as velocity, strain, and SR can be derived ( Figure 8 ). In addition, instantaneous velocity vectors can be calculated and superimposed on the dynamic images ( Figure 9 ). In contrast to DTI, analysis of these velocity vectors allows the quantification of strain and SR in any direction within the imaging plane. Depending on spatial resolution, selective analysis of epicardial, midwall, and endocardial function may be possible as well. STE has been validated for the assessment of myocardial deformation against sonomicrometry and clinically against DTI.
2D STE Image Acquisition
Speckle tracking is an offline technique that is applied to previously acquired 2D images. The use of low frame rates may result in a loss of speckles, which in successive frames may move out of plane or beyond the search area. On the other hand, high frame rates may be achieved by reducing the number of ultrasound beams in each frame, thereby reducing the spatial resolution and image quality. Therefore, although frame rates of 40 to 80 frames/sec have been used in various applications involving normal heart rates, higher frame rates are advisable to avoid undersampling in tachycardia.
The focus should be positioned at an intermediate depth to optimize the images for 2D STE, and sector depth and width should be adjusted to include as little as possible outside the region of interest. Any artifact that resembles speckle patterns will influence the quality of speckle tracking, and thus care should be taken to avoid these. For software packages that process single beats, data sampling should start ≥100 msec before the peak R wave of the first QRS complex and end 200 msec after the last QRS to allow correct identification of the QRS complex, because failure to do so may result in erroneous drift compensation. Apical foreshortening seriously affects the results of 2D STE, and should therefore be minimized. Similarly, the short-axis cuts of the left ventricle should be circular shaped to assess the deformation in the anatomically correct circumferential and radial directions.
2D STE Analysis of Myocardial Mechanics
Two-dimensional STE allows measurements of the above four parameters of myocardial mechanics by tracking groups of intramyocardial speckles ( d or v ) or myocardial deformation (ϵ or SR) in the imaging plane. STE-derived measurements of these parameters have been validated against sonomicrometry and magnetic resonance imaging.
Assessment of 2D strain by STE is a semiautomatic method, which requires manual definition of the myocardium. Furthermore, the sampling region of interest needs to be adjusted to ensure that most of the wall thickness is incorporated in the analysis, while avoiding the pericardium. When automated tracking does not fit with the visual impression of wall motion, regions of interest need to be adjusted manually until optimal tracking is achieved. For the left ventricle, because end-systole can be defined by aortic valve closure as seen in the apical long-axis view, this view should be analyzed first. If valve closure is difficult to recognize accurately (e.g., because of aortic sclerosis), a spectral Doppler display of LV outflow may be helpful.
Assessment of 2D strain by STE can be applied to both ventricles and atria. However, because of the thin wall of the atria and right ventricle, signal quality may be suboptimal. In contrast, all LV segments can be analyzed successfully in most patients. Feasibility is best for longitudinal and circumferential strain and is more challenging for radial strain.
The timing at which peak strain is measured is not uniform across publications. Peak strain can be measured as peak systolic strain (positive or negative), peak strain at end-systole (at time of aortic valve closure), or peak strain regardless of timing (in systole or early diastole). The time point to be used to measure peak strain in the assessment of systolic function depends on the specific question one wishes to answer.
Potential Pitfalls of 2D STE
Suboptimal tracking of the endocardial border may be a problem with STE. Another important limitation is its sensitivity to acoustic shadowing or reverberations, which can result in underestimation of the true deformation. Therefore, when strain traces appear nonphysiologic, signal quality and suboptimal tracking should be considered as potential causes. Tracking algorithms use spatial smoothing and a priori knowledge of “normal” LV function, which may erroneously indicate regional dysfunction or affect neighboring segmental strain values.
When using STE to measure LV twist, image quality of basal LV short-axis recordings can be a limitation. This is due in part to acoustic problems related to the depth of the basal part of the ventricle and to the wide sector angle that is necessary to visualize the entire LV base. Furthermore, measurements are complicated by out-of-plane motion when the base descends toward the apex in systole. Because LV rotation increases toward the apex, it is important to standardize the apical short-axis view. It is often easiest to find the correct circular apical short-axis view by tilting the probe from the apical four-chamber view rather than moving the probe in the apical direction from the parasternal short-axis view. The former also increases the chance of capturing a circular apical short-axis view when the endocardium nearly closes at end-systole.
Global strain might be inaccurate if too many segmental strain values are discarded because of suboptimal tracking. This is particularly true in localized myocardial diseases where strain values are unevenly distributed.
Strengths and Weaknesses of 2D STE
Both DTI and STE measure motion against a fixed external point in space (i.e., the transducer). However, STE has the advantage of being able to measure this motion in any direction within the image plane, whereas DTI is limited to the velocity component toward or away from the probe. This property of STE allows measurement of circumferential and radial components irrespective of the direction of the beam. Of note, however, is that STE is not completely angle independent, because ultrasound images normally have better resolution along the ultrasound beam compared with the perpendicular direction. Therefore, in principle, speckle tracking works better for measurements of motion and deformation in the direction along the ultrasound beam than in other directions. Similar to other 2D imaging techniques, STE relies on good image quality as well as the assumption that morphologic details can be tracked from one frame to the next (i.e., that they can be identified in consecutive frames), which may not be true when out of plane motion occurs. Because speckle tracking relies on sufficiently high temporal resolution, DTI may prove advantageous when evaluating patients with higher heart rates (e.g., during stress echocardiography) or if short-lived events need to be tracked (isovolumic phases, diastole, etc.).
A significant limitation of the current implementation of 2D STE is the differences among vendors, driven by the fact that STE analysis is performed on data stored in a proprietary scan line (polar) format, which cannot be analyzed by other vendors’ software. There are some implementations that operate on images stored in a raster (Cartesian) Digital Imaging and Communications in Medicine format, but there is only limited experience to date cross-comparing different vendors’ images. This issue needs further investigation before STE can become a mainstream methodology. There is currently a joint effort between the American Society of Echocardiography (ASE), European Association of Echocardiography (EAE), and the industry to address this issue.
2.3. Three-Dimensional (3D) STE
Although 2D STE is a useful technique, it has the intrinsic limitations of 2D imaging, such as the use of foreshortened views that affect the accuracy of the quantification of individual components of myocardial motion. In addition, the assumption that speckles remain within the 2D imaging plane and can be adequately tracked throughout the cardiac cycle may not always be valid, because of the complex 3D motion of the heart chambers. The inability of 2D STE to measure one of the three components of the local displacement vector is an important limitation, which affects the accuracy of the derived indices of local dynamics.
In contrast to 2D STE, which cannot track motion in and out of the imaging plane, the recently developed 3D STE can track motion of speckles irrespective of their direction, as long as they remain within the selected scan volume. Several recent studies showed that in individual patients, compared with 2D STE, 3D STE results in a more homogeneous spatial distribution of the measured parameters in normal ventricles. This finding is consistent with normal patterns of LV function and the fact that 3D STE can measure all three spatial components of the myocardial displacement vector ( Figure 10 ). As a result, 3D STE–based measurements of LV volumes were found to be in close agreement with magnetic resonance–derived reference values, and the levels of agreement were higher than those of 2D STE measurements obtained in the same patients, as reflected by higher correlation coefficients, smaller biases, and tighter limits of agreement.
Although 3D STE generates >3,000 vectors per volume and its temporal resolution is the same as the frame rate of real-time 3D data sets (typically 20–30 volumes/sec), its use was found to considerably reduce the examination time to one third of that for 2D STE. Furthermore, a significantly greater number of segments could be analyzed using 3D STE. This advantage of 3D STE stems from the fact that the entire left ventricle can be analyzed from a single volume of data obtained from the apical transducer position. These initial clinical results indicate that 3D STE may have important advantages over 2D STE, allowing a faster and potentially more complete and more accurate analysis of myocardial function, despite the relatively low temporal resolution.
3D STE Image Acquisition
Three-dimensional STE can be applied to 3D echocardiographic images acquired using a matrix-array transducer from the apical position in a wide-angled acquisition “full-volume” mode. In this mode, a number of wedge-shaped subvolumes are acquired over consecutive cardiac cycles during a single breath hold and stitched together to create one pyramidal volume sample. It is likely that 3D STE will be applicable to 3D data sets acquired in a single-beat mode, when this mode allows imaging at sufficiently high frame rates. Special care must be taken to include the entire LV cavity within the pyramidal volume, which may have a detrimental effect on temporal resolution.
3D STE Analysis of Myocardial Deformation
Pyramidal data sets are analyzed using dedicated, semiautomated 3D STE software. After anatomically correct, nonforeshortened apical views are identified at end-diastole and LV endocardial and epicardial boundaries are initialized, 3D endocardial and epicardial surfaces are automatically detected with manual adjustments as necessary. Subsequently, these borders are automatically tracked in 3D space throughout the cardiac cycle. To obtain regional information about LV motion and deformation, the ventricle is divided into 3D segments. Radial and longitudinal displacements and rotation, as well as radial, longitudinal, and circumferential strains, are automatically calculated for each segment over time. In addition, displacement in 3D space is calculated. Peak and time-to-peak values can also be obtained for each index similarly to 2D STE.
Potential Pitfalls of 3D STE
The major pitfall of 3D STE is its dependency on image quality. Random noise and relatively low temporal and spatial resolution affecting its ability to define the endocardial and epicardial boundaries. These issues likely affect the frame-to-frame correlation of local image features and contribute to suboptimal myocardial tracking. As with 2D STE, tracking quality should therefore be carefully verified and adjusted as necessary.
Strengths and Weaknesses of 3D STE
With the theoretical benefits gained by the addition of the third component of motion vector, which is “invisible” to either DTI or 2D STE, 3D STE promises to allow accurate assessment of regional ventricular dynamics. Nevertheless, it still requires rigorous validation and testing. On the downside, the much slower frame rates of 3DE compared with 2D STE may limit analysis of rapid events such as isovolumic contraction and relaxation. Future studies should assess the impact of these relatively low frame rates.
Another limitation is that although this methodology has been validated against sonomicrometry in animals, there is no true noninvasive “gold standard” technique that can be used in humans to validate regional ventricular function in three dimensions. As a result, most of the literature on this topic published thus far represents feasibility studies and potential advantages of 3D STE but does not establish the accuracy of the method. The clinical value of this new technology in a wide variety of clinical scenarios such as chamber volume measurements, evaluation of global and regional wall motion abnormalities ( Figure 11 ), and assessment of LV dyssynchrony in patients with heart failure remains to be determined in future studies.
Even more than 2D STE, 3D STE is currently implemented in ways specific to individual vendors. Although this methodology is in its infancy of development, it will be important to move toward vendor interchangeability.
2.4. Integrated Backscatter (IBS) Analysis
IBS analysis can be considered the first technique used for myocardial imaging, with initial studies being described as the method evolved in parallel with the development of 2D imaging more than three decades ago. IBS analysis describes a process of quantitative acoustic characterization of the myocardial structure. In this analysis, the power of the reflected signal in each scan line is quantified before the radiofrequency signal is demodulated to construct a real-time image. The IBS signal reflects the interaction between the incidental ultrasound wave and the structural heterogeneity in the myocardium and can be analyzed in either the time or the frequency domain.
IBS Signal Acquisition
The IBS signal is obtained from standard dynamic 2D images, usually in the parasternal long-axis view. However, images need to be saved in raw data format to allow IBS analysis before the image is processed.
IBS Analysis of Myocardial Dynamics
This analysis is performed by dedicated software that may be used for two major purposes. Variation in backscatter during the cardiac cycle is thought to reflect the crossover of actin and myosin within the myofibrillar structure. This process results in changes of reflectivity, and the resulting cyclic variation has been shown to correlate with myocardial strain. The problem is that because of the myocardial anisotropy (directional nonuniformity of myofibers), the pattern of this variation differs from wall to wall and view to view, such that specific normal ranges need to be documented for each wall and each view. Nonetheless, the signal has been used as a noninvasive marker of contractility, for example in the assessment of myocardial viability. The second measurement approach relates to the comparison of myocardium with other tissues to document the reflectivity of the myocardium ( Figure 12 ). In the absence of a frame of reference, this parameter would be influenced by gain settings and patient habitus, so the provision of this material requires calibration with an intrinsic frame of reference (calibrated IBS), such as the pericardium (which is brighter than myocardium) or blood pool (which is darker).
The results of clinical studies have been described at length in previous review articles. Normal ranges of cyclic variation in IBS of the septum and posterior wall vary from 4.5 to 6.0 dB. Dilated cardiomyopathy has been associated with obstruction of cyclic variation, corresponding to areas of reduced function. Likewise, reduced cyclic variation has been documented in the setting of acute myocardial infarction. In viable myocardium, residual cyclic variation is detected even though the tissue may appear akinetic. Cyclic variation in IBS is also reduced in early myocardial disease, for example because of diabetes or hypothyroidism. Likewise, calibrated IBS has been used as a marker of fibrosis in a variety of cardiac conditions, including hypertensive heart disease and hypertrophic cardiomyopathy. Biopsy studies have been performed to validate the presence of fibrous tissue in these settings. Also, IBS can identify an increase in atrial degeneration that might predict the occurrence of atrial fibrillation before LA dilation.
Potential Pitfalls of IBS Analysis
In the image setup, care needs to be taken with the output power settings to ensure that the signal is not saturated. This is particularly a problem when using video signal, rather than the radiofrequency signal, because the relationship between signal intensity and brightness is nonlinear at the upper and lower extremes of signal intensity. The ultrasound beam should be perpendicular to the interrogated wall, and measurements should be performed within the myocardium while avoiding the endocardium, because the blood-tissue interface is much brighter than the intramyocardial signal and can lead to overestimation of the IBS signal.
Strengths and Weaknesses of IBS Analysis
The attraction of this application is that myocardial texture may be analyzed from standard grayscale images. Potentially, this method could be used to quantify tissue characteristics independent of the usual parameters of LV shape and function. However, there are a variety of weaknesses. The technique is susceptible to poor image quality and signal noise. Measurements are generally restricted to the anteroseptal and posterior segments in the parasternal views. Although other segments and views may be imaged, the user should be aware that normal ranges are less well defined and that the variability of the signal is greater, related to angulation issues.
The long history of this technique compared with its rare clinical use tells its own story in relation to its difficulty. This procedure is technically demanding, subject to artifacts related to the presence of other myocardial reflectors, image settings, and the exact location of the sample volume. In the era of strain measurement, there is little to recommend the ongoing measurement of cyclic variation as a marker of contractility. Calibrated backscatter still has value as a marker of fibrosis, but this appears to be most effective in more severe disease. Therefore, this methodology remains more of a research instrument than a clinical tool.
3. Physiologic Measurements of Left Ventricular Function
3.1. LV Architecture and Vectors of Myocardial Deformation
Knowledge of the cardiac micro and macro architecture is useful in understanding the relative contributions of different myocardial layers to the 3D components of myocardial deformation. This information is important for optimizing motion analysis using DTI and STE.
Several studies have explored the 3D deformation of the ventricular tissue, describing myocyte arrangements as a continuum of two helical fiber geometries. The subendocardial region shows a right-handed helical myofiber geometry, which changes gradually into a left-handed helical geometry in the subepicardium. Thus, the longitudinal axis of cardiac myofiber sheets rotates continuously. In the subendocardium, the fibers are roughly longitudinally oriented, with an angle of about 80° with respect to the circumferential direction. The angle decreases toward the midwall, where the fibers are oriented in the circumferential direction (0°), and decreases further to an oblique orientation of about −60° in the subepicardium ( Figure 13 A).
This structural anisotropy of the LV wall affects the propagation and backscatter of ultrasound waves and the appearance of cardiac tissue in echocardiographic images. Greater backscatter and brighter speckles are seen when myofibers and the ultrasound beam are perpendicular rather than parallel. For example, in the apical four-chamber view, bright speckles in the middle of the interventricular septum represent the location of the middle layer of circumferentially oriented fibers that are perpendicular to the beam ( Figure 13 B). In short-axis views, bright speckles are seen in the anterior and posterior segments where myofiber sheets are perpendicular to the beam, while marked attenuation occurs within the septum and the lateral wall, where the myofiber sheets are relatively parallel to the scan lines ( Figure 14 ).
The above myocardial structure broadly determines the components of myocardial deformation. The subendocardial region contributes predominantly to the longitudinal mechanics of the left ventricle, whereas the midwall and the subepicardium contribute predominantly to the rotational motion.
Longitudinal and Circumferential Mechanics
During preejection, reshaping of LV geometry causes simultaneous shortening and stretch of the early and the late activated regions, respectively. Thus, shortening of subendocardial fibers is accompanied by simultaneous subepicardial fiber stretching. Segmental stretch may also be seen in the late activated regions of the subendocardium, particularly near the basal posterolateral region, which is the last area of the ventricle to activate. The onset of longitudinal and circumferential shortening therefore shows substantial transmural and apex-to-base heterogeneity.
Subendocardial and subepicardial layers shorten concurrently during ejection. The magnitude of circumferential strains during ejection exceeds that of longitudinal strains. Furthermore, longitudinal and circumferential shortening strains during ejection show a small apex-to-base gradient, such that successive shortening strains are higher at apical and mid segments compared with the LV base.
The postejection period also shows significant heterogeneity in the onset of myofiber relaxation. Lengthening of subepicardial fibers is accompanied by shortening of subendocardial fiber sheets. This transient heterogeneity accounts for physiologic longitudinal postsystolic shortening of myocardial segments recorded in normal human subjects.
Radial Mechanics
Continuum mechanics would suggest that shortening in the longitudinal and circumferential direction would result in thickening in the radial direction for conservation of mass. However, LV wall thickening is not a result of simple shortening of individual myocytes but an effect of shearing of groups of myocytes across one another. One of the principal purposes of cardiac shearing deformation lies in amplifying the 15% shortening of myocytes into >40% radial LV wall thickening, which in turn results in a >60% change in LV ejection fraction (EF) in a normal heart. Because the degree of shearing increases toward the subendocardium, higher thickening strains are seen at the subendocardium in comparison with the subepicardium. This difference does not reflect a difference in contractility between wall layers but is a consequence of geometry and tissue incompressibility. Transmural heterogeneity in the timing of wall thickening mechanics is also seen during the preejection and postejection phases of the cardiac cycle.
Twist Mechanics
The helical nature of the heart muscle determines its wringing motion during the cardiac cycle, with counterclockwise rotation of the apex and clockwise rotation of the base around the LV long axis, when observed from the apical perspective.
In a normal heart, the onset of myofiber shortening occurs earlier in the endocardium than the epicardium. During preejection, subendocardial shortening and subepicardial stretch contribute to a brief clockwise rotation of the LV apex. During ejection, the activation and contraction of the subepicardial region with a larger radius of arm of moment produces higher torque to dominate the direction of rotation, resulting in global counterclockwise LV rotation near the apex and clockwise rotation near the LV base. Twisting and shearing of the subendocardial fibers deform the matrix and result in storage of potential energy.
Subsequent recoil of twist, or untwist, which is associated with the release of restoring forces contributes to diastolic suction, which facilitates early LV filling. The onset of myofiber relaxation occurs earlier in epicardium than endocardium. Thus, at early diastole, both subepicardial lengthening and subendocardial shortening facilitates recoil in the clockwise direction. Nearly 50% to 70% of LV untwisting occurs within the period of isovolumic relaxation, while the rest is completed during early diastolic filling phase. One manifestation of this is that during systole, twisting occurs simultaneously with long-axis and radial shortening, while during diastole, untwisting distinctly precedes lengthening and expansion, a phenomenon that is even more marked with exercise. This leads to a linear relation between twist and LV volume during ejection with a nonlinear curve in diastole.
Components of Myocardial Deformation and Transmurality of Disease
In general, longitudinal LV mechanics, which are predominantly governed by the subendocardial layer, are the most vulnerable and most sensitive to the presence of myocardial disease. If unaffected, midmyocardial and epicardial function may result in normal or nearly normal circumferential and twist mechanics with relatively preserved LV pump function and EF. However, compromised early diastolic longitudinal mechanics and reduced and/or delayed LV untwisting may elevate LV filling pressures and result in diastolic dysfunction. On the other hand, an acute transmural insult or progression of disease results in concomitant midmyocardial and subepicardial dysfunction, leading to a reduction in LV circumferential and twist mechanics and a reduction in EF. Thus understanding the layer-based contributions to the components of cardiac deformation helps in estimating the transmural disease burden correctly and provides pathophysiologic insight into the mechanisms of LV dysfunction.
3.2. Clinical Use of LV Displacement, Velocity, Strain, and SR
There is a wealth of literature on the use of the displacement and deformation indices of myocardial dynamics in multiple disease states. The following is a brief summary of the existing body of knowledge.
Normal Values
Normal values of the parameters of LV myocardial mechanics vary depending on the specific LV wall and the specific 3D component of each index. For both DTI and STE, longitudinal measurements are more robust than radial ones. Because the apical window allows interrogation of all LV myocardial segments, most available clinical data pertain to longitudinal deformation. Longitudinal velocities in the lateral wall are higher than in the septum. There is also a base-to-apex gradient, with higher velocities recorded at LV base than near the apex. Minor differences are seen between LV segments for DTI-derived strain and SRs. STE-derived measurements generally show higher values in the apical segments than DTI. Within a segment, higher velocities and strains are usually recorded from the subendocardium than from the subepicardium. Velocities and deformation parameters are also affected by age and loading conditions. In a recent study in a large European population, the lower limits of normal range with the Doppler method were found to be 18.5% and 44.5% for longitudinal and radial strain and 1.00 and 2.45 sec −1 for longitudinal and radial SR. Normal deformation values vary among publications and importantly depend on the brand of imaging equipment, which does not use the same algorithms to process measured data across vendors. Moreover, loading conditions and heart rate need to be taken into account when interpreting all functional data.
Published Findings
Estimation of LV Filling Pressures
LV filling pressures obtained by cardiac catheterization show good correlation with the ratio of the mitral inflow E velocity to DTI-derived mitral annular wave (E/e′). E/e′ (lateral) ≥12 and E/e′ (septal) ≥15 are correlated with elevated LV early diastolic pressure, and E/e′ (lateral, septal, or average) <8 is correlated with normal LV early diastolic pressure.
Subclinical Disease
Strain and SR analysis increase sensitivity in detecting subclinical cardiac involvement in conditions such as amyloidosis, diabetes, and hypertensive heart disease, as well as changes in LV function after cancer treatment, because e′ velocity is reduced in patients with all these conditions.
Constrictive Versus Restrictive Physiology
In the absence of myocardial disease, e′ velocities typically remain normal in patients with constrictive pericarditis (usually >8 cm/sec). In contrast, intrinsic myocardial abnormalities characteristic of restrictive cardiomyopathy result in impaired relaxation and reduced e′ velocities.
Athlete’s Heart Versus Hypertrophic Cardiomyopathy
The presence of brisk e′ velocities is seen in athletes’ hearts in contrast to the reduced e′ velocities seen in individuals with hypertrophic cardiomyopathy.
Mitral and Tricuspid Annular Motion
The mitral and tricuspid annuli are anatomic structures that may be distinctly visualized by 2D echocardiography in almost all patients, irrespective of endocardial visualization. As a result, annular longitudinal displacement can be accurately assessed in the majority of patients.
Myocardial Strain and SR
In disease states, myocardial deformation patterns may either remain comparable with normal, but reach lower peak values, or show striking changes as the disease progresses.
Coronary Artery Disease
Changes in strain not only facilitate recognition of ischemic myocardium during stress echocardiography ( Figure 15 ) but also may provide prognostic information. Furthermore, assessment of cardiac strain helps in defining the transmural extent of myocardial infarction and the presence of viable myocardium.
Cardiomyopathy
Cardiac strain and SRs may be reduced in cardiomyopathy and potentially could be used for monitoring disease progression and the impact of therapeutic interventions. DTI and speckle-tracking echocardiographic measurements are helpful in quantifying LV dyssynchrony. However, there is currently a lack of consensus on how LV dyssynchrony indices should be measured in clinical practice.
Congenital Heart Disease
Several studies have recently tested the use of DTI and STE to assess myocardial deformation and strain in children, both normal and with congenital abnormalities. However very little is known to date as far as clinical usefulness of these techniques in the context of congenital heart disease.
Unresolved Issues and Research Priorities
A growing body of evidence suggests that the assessment of LV deformation by Doppler or speckle-tracking techniques provides incremental information in clinical settings. The areas that hold the greatest promise for potential applications include assessment of myocardial ischemia and viability (see below), detection of subclinical heart disease, and the serial assessment of different cardiomyopathies. One of the major challenges, however, is the rapid pace of technological growth, which has resulted in a variety of software packages and algorithms. Future clinical trials therefore need to include standardization of nomenclature, steps in data acquisition, and optimal training to reduce data variability.
Summary and Recommended Indications
Clinical applications of DTI or STE derived myocardial displacement, velocity, strain, and SR measurements are gradually becoming established as tools for the assessment of LV diastolic function but still require further refinements. DTI-derived mitral annular velocities by pulsed-wave Doppler are recommended for the routine clinical evaluation of LV diastolic function, as described in detail below in the section 3.5. STE-derived and DTI-derived strain parameters are comparable, but STE has advantages with regard to ease of application and analysis and for data reproducibility. For both techniques, the accuracy of measurements, however, depends on image quality and the accuracy of tracking. In expert hands, strain and SR parameters can improve accuracy and prognostic value of stress echocardiograms. Further technical development and standardization of methodology are necessary before additional clinical application can be recommended.
3.3. LV Rotation
LV rotation or twisting motion has an important role in LV systolic and diastolic function. Although LV rotational deformation can be quantified using color DTI with high temporal resolution, this method is technically demanding and has not achieved widespread adoption. In contrast, multiple recent studies have demonstrated that 2D STE represents a clinically feasible alternative to color DTI in evaluation of myocardial rotation and twist mechanics in the majority of patients.
Normal Values
Normal values for LV rotation and net twist angle show variability depending on the technique used for measurement, the location of the region of interest in the subendocardium or the subepicardium, the age of the subject, and the loading hemodynamics of the ventricle. A recent study of a large group of healthy volunteers reported a mean value of peak LV twist angle as 7.7 ± 3.5°. Peak LV twist angle was significantly greater in subjects aged >60 years (10.8 ± 4.9°) compared with those aged <40 years (6.7 ± 2.9°) and even those aged 40 to 60 years (8.0 ± 3.0°). The increase in LV twist angle can be explained by less opposed apical rotation, resulting from a gradual decrease in subendocardial function with aging. Worsening of diastolic relaxation and reduced early diastolic suction is, however, associated with reduction in the rate and magnitude of untwisting. In a study of patients from infancy to middle age, it was noted that twist increases from 5.8 ± 1.3° in infancy to 6.8 ± 2.3° in grade school, 8.8 ± 2.6° in the teenage years, and 13.8 ± 3.3° in middle age. Apical rotation was fairly constant in childhood, with basal rotation transition from counterclockwise in infancy to the adult clockwise rotation, causing most of the increase in twist in childhood. Subsequently, the twist increases, mainly because of a gradual increase in apical rotation with age.
Published Findings
Because apical rotation accounts for most of LV twist, apical wall motion abnormalities significantly impair peak LV twist parameters. This may be manifested by (1) a reduction of initial clockwise twist during early systole, (2) augmentation of peak counterclockwise twist, and (3) reduction in LV untwisting during early diastole ( Figure 16 ). Major findings reported in published studies are described below and summarized in Table 1 .
LV twist | Untwisting | Time to peak untwisting | |
---|---|---|---|
Heart failure | |||
With preserved LV EF | → or ↑ | → or ↑ | Delayed |
With reduced LV EF | ↓ | ↓ | Delayed |
Coronary artery disease | |||
Subendocardial MI | → | → | Delayed |
Transmural MI | ↓ | ↓ | Delayed |
Aortic stenosis | ↓ | → or ↑ | Delayed |
LV hypertrophy | |||
Hypertension | → or ↑ | ↓ | Delayed |
HCM | Variable | Variable | Delayed |
Dilated cardiomyopathy | ↓ | ↓ | Delayed |
Pericardial disease | ↓ | ↓ | No data provided |
Heart Failure Syndromes
In early stages of heart failure, diastolic dysfunction is associated with relatively preserved or even higher LV net twist angle in the presence of normal EF. The onset of untwisting and peak untwisting in early diastole, however, is significantly delayed and can be further unmasked during exercise. Patients with heart failure and reduced EF show progressive reduction of LV twist angle and untwisting velocities. However, in patients with heart failure and preserved systolic function, peak untwisting is usually normal but may be delayed in a subset of patients.
Coronary Artery Disease
Although LV longitudinal strain is attenuated in the presence of subendocardial perfusion deficit, LV circumferential deformation and twist may remain unaltered in ischemic myocardium. Similarly, patients with subendocardial infarcts and preserved EF show reduced radial and longitudinal strain, although LV circumferential strains and twist mechanics remain relatively preserved. In contrast, a larger transmural infarction is associated with reduction in LV systolic twist angle and diastolic untwisting velocity, which correlates with the reduced EF.
Aortic Valve Disease
Net LV twist angle is significantly increased in aortic valve stenosis, although diastolic apical untwisting is prolonged in comparison with normal subjects. After aortic valve replacement, LV twist angle normalizes.
Mitral Valve Disease
Changes in LV twist angle have also been studied in patients with mitral regurgitation. Chronic mitral regurgitation is associated with complex LV adaptive remodeling and eccentric hypertrophy. The effect of chronic mitral regurgitation on twist probably depends on the extent of subclinical LV systolic dysfunction. Peak untwisting velocity in mitral regurgitation remains normal but correlates negatively with end-systolic dimension and regurgitant volume, suggesting that peak untwisting velocity, like peak systolic twist, depends on the stage of the disease. This confirms the important effect of LV end-systolic volume on LV untwisting, because LV end-systolic volume was found to be an important determinant of peak untwisting velocity, irrespective of EF.
Cardiomyopathy
In dilated cardiomyopathy, the amplitude of peak LV systolic twist angle is impaired in proportion to global LV function. This reduction in LV twist angle is accounted for by marked attenuation of LV apical rotation, whereas basal rotation may be spared. In some cases, rotation of the apex may be abruptly interrupted, such that in the major part of systole, the LV base and apex rotate clockwise together in the same direction. For patients undergoing cardiac resynchronization therapy (CRT), an immediate improvement in LV twist angle has been reported to predict LV reverse remodeling at 6-month follow-up.
Dilated Versus Hypertrophic Cardiomyopathy
In contrast to dilated cardiomyopathy, patients with hypertrophic cardiomyopathy show variability in the extent of LV twist and untwisting, depending on the extent and distribution of hypertrophy and obstruction. Patients with LV hypertrophy due to systemic hypertension, however, show relatively preserved LV twist mechanics, although LV untwisting velocities, particularly during isovolumic relaxation, are both attenuated and delayed. The marked endocardial dysfunction with relative sparing of epicardial function leads to abnormal longitudinal mechanics, with relative sparing of circumferential and twist mechanics in restrictive cardiomyopathy.
Pericardial Disease
Like constrictive pericarditis, pericardial diseases show predominant impairment of circumferential and twist mechanics, while relatively sparing subendocardial longitudinal mechanics.
Diastolic Function
During systole, a significant amount of elastic energy is stored in the myocyte and the interstitium as torsion. The earliest mechanical manifestation of diastole is an abrupt untwisting that is largely completed before the mitral valve opens. This untwisting helps establish a base-to-apex intraventricular pressure gradient (IVPG), or diastolic suction, in early diastole that assists in the low-pressure filling of the heart. A study of normal controls and patients with hypertrophic cardiomyopathy examined the relationship between untwisting rate and IVPG at rest and with low-level (heart rate about 100 beats/min) exercise. IVPG has been shown to be calculable by applying the Euler equation to color M-mode transmitral flow propagation data in dogs and humans. In both normal controls and patients with hypertrophic cardiomyopathy, there was a linear relationship between ventricular untwisting rate and peak diastolic suction gradient ( r = 0.72). This mirrors another exercise study that showed that the best determinant of maximal myocardial oxygen consumption in normal subjects and in patients with heart failure was the ability to augment diastolic suction with exercise, while an animal study confirmed that untwisting was directly related to IVPG and inversely related to the ventricular relaxation time constant τ.
Unresolved Issues and Research Priorities
The lack of standardization of imaging planes and different speckle-tracking algorithms among vendors make it difficult to make comparisons or establish normal values for LV twist with high levels of confidence. A multicenter study in a large number of normal subjects with different ultrasound machines is required to resolve this issue. Also, the development of 3D STE is likely to allow standardization of LV planes used for assessing twist torsion measurements. Moreover, population samples representing diseases affecting cardiac function should be studied to determine the diagnostic power and abnormal ranges of LV twist values.
Another issue that needs to be clarified relates to the definitions of “rotation,” “twist,” and “torsion.” One may find in the recent literature that these terms are sometimes used interchangeably. Because the mathematical definitions clearly differentiate among these three entities, it is important that they are used correctly, as defined in section 1.1.
Summary and Recommended Indications
Despite the growing evidence in support of clinical implications of LV twist measurements using 2D STE, routine clinical use of this methodology is not recommended at this time.
3.4. LV Dyssynchrony
Echocardiographic approaches to imaging dyssynchrony encompass several techniques, including M-mode, 2D, DTI ( Figures 17 and 18 ), STE, and 3D echocardiography. To date, several studies have examined the feasibility of using these techniques for predicting response to CRT. Current guidelines define the indications for CRT on the basis of clinical findings (heart failure symptoms and New York Heart Association class), LV function (EF), and electrocardiographic findings (QRS width) only. However, approximately one third of patients treated with CRT do not respond to this treatment with improvement in LV function, reflecting the clinical need for better patient selection and means of therapy optimization.
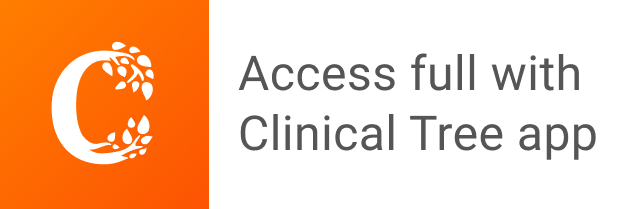