Since the late 1970s, perhaps the most important change in the practice of clinical pediatric cardiology has been the introduction of cross-sectional echocardiography and its subsequent technical improvements. Echocardiography currently allows highly accurate diagnosis of nearly all morphologic abnormalities, for the most part making invasive diagnostic techniques obsolete. Cardiac catheterization and angiography are no longer a routine investigation in most patients prior to cardiac surgery, as it used to be a few decades ago. Indeed, the main indication for such an investigation, apart from the purposes of assessing hemodynamics and intervention, is the display of structures beyond echocardiographic visualization, such as peripheral pulmonary arteries, distal coronary arteries, and more rarely complex abnormalities of systemic and pulmonary venous return. Even so, for many of these indications, magnetic resonance and computerized tomographic imaging have replaced cardiac catheterization. All pediatric cardiologists, cardiac intensivists, and pediatric cardiac surgeons should therefore be familiar with echocardiographic imaging and diagnosis. More recently point-of-care echocardiography has been introduced in the neonatal intensive care unit for specific diagnostic applications such as monitoring ductal patency and pulmonary arterial hypertension of the newborn. The increased availability of handheld echo machines has expanded their use in the pediatric emergency department, potentially allowing faster diagnosis of cardiac dysfunction and pericardial effusion in life-threatening conditions. This chapter is devoted to the principles of cross-sectional and Doppler echocardiography as applied in clinical practice, as well as more advanced applications, such as tissue Doppler techniques, myocardial strain imaging, and three-dimensional echocardiography.
Physical Principles of Ultrasonic Imaging
This chapter includes a limited introduction to the physical principles of ultrasound because some background knowledge is relevant for optimization of images in clinical practice. Those interested in more details on the physics of imaging should consult more comprehensive reviews or specialized textbooks of echocardiography.
Physical Properties of Ultrasound
An acoustic wave is a mechanical wave causing local compression and refraction while traveling through a medium. Ultrasonic waves are longitudinal sound waves with a frequency greater than 20 kHz, the highest frequency that can be detected by the human ear. The waves are generated and detected by a piezoelectric crystal, which deforms under the influence of an electrical field. The velocity of a sound wave is dependent upon the density and stiffness of the medium. Stiffness is the hardness or resistance of the material to compression. Density is the concentration of matter. Increase in stiffness also increases speed, whereas an increase in density decreases speed. Transmission of ultrasonic waves is slow in air or gasses but fast in solids. In the body tissues, the velocity of sound is relatively constant, at 1540 m/s. The frequency represents the numbers of cycles occurring in each second of time and is expressed in hertz (Hz), with 1 Hz corresponding to one cycle per second. The wavelength corresponds to the length over space over which one cycle occurs. The amplitude reflects the strength divided by the intensity of a soundwave and is expressed in decibels. The velocity of transmission, the frequency, and the wavelength are related by the formula c = f × λ , where c is the speed of sound through the medium, f is the frequency of the wave, and λ is the wavelength.
The range of frequencies used for medical imaging is from 2 to 12 megahertz (mHz). Corresponding wavelengths are in the range 0.8 to 0.13 mm. Wavelength is directly related to spatial resolution because two structures need to be separated by more than one wavelength to be resolved and imaged as two separate structures. This relationship explains why a higher-frequency probe has a better spatial resolution compared with a lower-frequency probe. When sound travels through a heterogeneous medium, different interactions occur, such as reflection, attenuation, refraction, and scattering. Reflection occurs at a boundary or interface between two mediums having a different acoustic density. The difference in acoustic impedance between the two tissues causes reflection of the sound wave in the direction of the transducer. Reflection from a smooth or specular interface between tissues causes the sound wave to return to the transducer. Irregular interfaces will cause scatter in different directions. The ultrasonic image is created based on the reflected waves as received by the transducer. Refraction is the phenomenon that the ultrasound wave, which passes through the tissue, is refracted based on the incidence of the beam. The incidence is oblique when the direction of the sound beam is not at a right angle to the boundary of the two mediums. This same phenomenon explains why a straight pencil that sits in a glass of water appears to have a bend in it. Attenuation is the loss of sonic energy as sound propagates through a medium. It is produced by the absorption of the ultrasonic energy by conversion to heat, as well as by reflection and scattering. The deeper the wave travels in the body, the weaker it becomes. The amplitude and strength of the wave decrease with increasing depth. Overall attenuation is dependent upon the frequency, such that ultrasound with lower frequencies penetrates more deeply into the body than ultrasound with higher frequencies. The shorter the wavelength (i.e., the higher the frequency), the faster the particle motion and the larger the viscous effects. Higher-frequency waves will thus attenuate more and penetrate less deep into the tissue. Probes with lower frequencies produce better penetration but, as mentioned previously, have lower spatial resolution. Probes with higher frequencies have lower penetration but higher spatial resolution. For pediatric echocardiography, high spatial resolution is generally required to visualize small cardiac structures; therefore imaging with the highest-possible frequency probe is recommended.
Attenuation also depends on acoustic impedance and mismatch in impedance between adjacent structures. Because air has a very high acoustic impedance, any air between the transducer and the cardiac structures of interest results in substantial attenuation of the signal produced. This is avoided during transthoracic examinations by use of water-soluble gel to form an airless contact between the transducer and the skin. The air-filled lungs are avoided by careful positioning of the patient and by the use of acoustic windows that allow access of the ultrasonic beam to the cardiac structures without the need to pass through intervening lung tissue. Attenuation causes decrease in amplitude as the wave passes through the tissue. In most ultrasonic systems, this is corrected for by automatic compensation. Further manual compensation in terms of gain in depth or time can be achieved by changing controls on the machine, which correct for the automated attenuation at specific depths of image. Current ultrasound machines provide tools for automated image optimization that makes the need for manual adjustments less frequent.
Production of Images
Each ultrasonic pulse, encountering numerous interfaces, gives rise to a series of reflected echoes returning at different time intervals corresponding to their depths. In this way, each pulse from the ultrasonic crystal generates a radiofrequency signal that represents the amplitude of the reflected ultrasound wave as a function of time. The signal detected by the transducer is typically electronically amplified. The amount of amplification has a preset value but can be modified on an ultrasound system by using the “gain” button (typically the largest button on the operating panel). Importantly, the overall gain will amplify both the signal and potential measurement noise and will thus not affect the signal-to-noise ratio. The returned signal is processed so that the radiofrequency signal is converted into an image. The envelope of the radiofrequency signal is detected (demodulation) and is subdivided as a function of time in small intervals (pixels). Each pixel is attributed a gray-scale number, defined by the local amplitude of the signal, ranging between 0 and 255 (8-bit image). “0” represents “black,” and “255” represents “white.” Encoding can be changed to color maps (e.g., bronze) by changing from grayscale and selecting different color maps. This can improve image visualization in the case of poor imaging windows. Current scanners allow 12 and 16 bit encoding 4096 or 65,536 gray/color levels. The contrast of the images can further be changed by adjusting the compression or dynamic range, which results in changing the representation of the images but does not affect the acquisition. A low dynamic range or compression will result in more black and white images, whereas high compression or dynamic range adds a lot of gray values to the images.
For M-mode (motion mode) acquisition, the ultrasound beam is transmitted into the same direction for each transmitted pulse. M-mode represents one line of information displayed on the vertical axis, with time on the horizontal axis. Its advantage is the very high temporal resolution. The frequency of repetition of the pulse producing a typical M-mode trace is more than 1000 frames per second. Conventional cross-sectional echocardiography or B-mode imaging (“brightness mode”) depends on the construction of an image using multiple individual lines of information sent out in slightly tilted directions. Typically 64 or 128 lines of information are required to produce one image or frame. Multiple frames are constructed in real time each second, the limiting factor being the time necessary for the echoes from each pulse to return to the transducer. Consequently, at depths of 5 to 15 cm, it is possible to achieve frame rates of 28 to 50 per second. Modern systems are capable of manipulating the frame rate by sending out different pulses at different times, but in general increasing the frame rate will reduce the quality of the image, and hence its spatial resolution. Frame rates and thus spatial resolution can be increased by reducing the imaging sector width (less lines and thus less time for construction of each frame). For pediatric imaging, optimizing both spatial and temporal resolution is a trade-off, and depending on the information required, priority can be given to either. More recently, high-frame rate or ultrafast ultrasound has been introduced with frame rates up to 5000 to 10,000 frames/s. Modern phased-array probes are built based on an array of piezoelectric crystals and not on mechanical motion of one single piezoelectric element. By introducing specific time delays between the excitation of different crystals in the array, the ultrasound wave can be sent in a specific direction without mechanical motion of the transducer. The received RF signal for a transmission in a particular direction is then simply the sum of the signals received by the individual elements. These individual contributions can be filtered, scaled, and time-delayed separately before summing. This process is referred to as beam-forming and is a crucial element for obtaining high-quality images. There same principle is applied in three-dimensional arrays.
Second Harmonic Imaging
When the amplitude of the transmitted wave becomes significant, the shape of the ultrasound wave will distort during propagation. This wave distortion typically generates harmonic frequencies (i.e., integer multiples of the transmitted frequency). So, for example, transmitting a 1.7-MHz ultrasound pulse will result in the generation of frequency components of 3.4, 5.1, 6.8, 8.5 MHz, and so on. These harmonic components will grow stronger with propagation distance. The ultrasound scanner can be set up to receive only the second harmonic component through filtering of the received radiofrequency signal. Such an image typically has a better signal-to-noise ratio by avoiding clutter noise due to (rib) reverberation artifacts and increases image resolution deeper in the tissue. Thus harmonic image can improve image quality in patients with poor acoustic windows and limited penetration. However, it has intrinsically poorer axial resolution. Harmonic imaging has become the default cardiac imaging mode for adult scanning on many systems. In younger infants, it is unnecessary to use harmonic imaging and it needs to be avoided because it reduces image resolution. Switching between conventional and harmonic imaging is done by changing the transmit frequency of the system.
Quality of Images
Image quality refers to the resolution of the imaging system. Spatial resolution refers to the capacity of the system to resolve small structures. Contrast resolution is the ability of the system to distinguish differences in the density of the soft tissues. Temporal resolution refers to the capacity of the system to resolve differences in time.
Spatial Resolution
This can be defined as the combination of axial and lateral resolution. Axial resolution is the capacity of the ultrasonic system to distinguish how close together two objects can be along the axis of the beam, yet still be distinguished as two separate objects. As mentioned previously, wavelength affects axial resolution and is improved by increasing the ultrasound frequency. Axial resolution is much higher compared with lateral resolution, which is the capacity of the system to resolve two adjacent objects that are perpendicular to the axis of the beam as separate entities. The width of the beam affects the lateral resolution: the wider the beam, the lower the lateral resolution. This is influenced by the focal zone, which is the depth of the smallest beam width. The near field is the zone between the transducer and the focal zone, and the far field is the region beyond the focal zone. Optimizing the focus at a certain depth improves lateral resolution. The limits of the focal zone are determined by the size and frequency of the transducer. Small transducers focus well in the near field, whereas large transducers perform better in the far field. The width of the beam is also influenced by the frequency of the transducer, with higher-frequency probes having a better lateral resolution compared with those that have a low frequency. However, probes with higher frequency suffer from their limited ability to penetrate into the tissue. Line density can also be improved by decreasing the sector width, resulting in better lateral resolution but more limited image sector.
Temporal Resolution
To image fast-moving structures, such as valve leaflet motion, frame rate optimization is highly important. The eye generally can see only 25 frames per second, providing a temporal resolution of approximately 40 ms. The temporal resolution is limited by the sweep speed of the echo beam, which in turn is limited by the speed of sound because the echo from the deepest part of the image has to return before the next pulse is sent out at a different angle in the neighboring beam. The speed of the sweep can be increased by reducing the number of beams, or increasing the beam width in the sector, by using the frame rate control, or by decreasing the width of the sector. The first option decreases the lateral resolution, whereas the second decreases the image field. Temporal resolution, therefore, cannot be increased without a trade-off, due to the physical limitations of echocardiography. Newer technology such as plane wave imaging can overcome this limitation but remains an emerging technology not routinely available in the clinic.
Ultrasonic Imaging Artifacts
There are many potential sources for artifacts in echocardiography. For interpretation of the images, it is important to know and recognize them.
- ■
Dropout of parallel structures: When structures are parallel to the ultrasonic beam, there is very little reflection caused by the structure, resulting in dropout. A typical example is the atrial septum when viewed from the apex.
- ■
Acoustic shadowing: Transmission of the ultrasonic beam through the tissue is influenced by the presence of tissue with very high density. Typical examples are prosthetic valves, devices, catheters, or calcifications. These structures can make it impossible to view structures behind them.
- ■
Reverberations can occur with lateral spread of high-intensity echoes. Bright echoes can have considerable width. A lot of reverberations originate from the interaction between the transducer and the ribs.
- ■
Mirror imaging. This artifact appears as a display of two images, one real and one artifactual, and is due to the sound beam interacting with a strong reflector.
- ■
Ring down or comet tail. The ring down artifact comes from gas bubble in a fluid medium. The comet tail originates from highly reflective structures, such as surgical clips.
Doppler Echocardiography
The Doppler principle is the frequency shift caused in ultrasonic waves as they are reflected by a moving reflector. The frequency of reflected waves increases as the target approaches the receiver and decreases as it moves away from the observer. A typical example is the sound of an ambulance siren, which has a higher pitch sound when moving towards the observer, and a lower pitch sound when moving away. When initially applied to echocardiography, the Doppler technique was used to measure the velocities of blood pools. A Doppler shift is caused by interaction of the ultrasonic beam with a moving pool of red blood cells. The Doppler shift depends on the velocity and direction of the flowing blood, the angle between the beam and the flow, and the velocity of sound within in the tissues. This is expressed in the Doppler formula:
Fd=2F0Vcosθc
The observed frequency shift is several kilohertz in magnitude and produces an audible signal that can be electronically processed and displayed graphically. Because different velocities are present within the ultrasonic beam for any given time instance during the cardiac cycle, a range of Doppler frequencies are typically detected. Thus a spectrum of Doppler shifts is measured and displayed in the spectrogram, hence the term spectral Doppler as often encountered in the literature. The Doppler-shifted frequencies usually undergo fast-Fourier transformation, converting the original Doppler waveform into a spectral display with velocity on the vertical axis, time on the horizontal axis, and amplitude as shades of gray. Conventionally, Doppler signals from blood moving toward the transducer are displayed above the baseline. Similarly, when blood flows away from the transducer, the display is below the baseline. When the Doppler shift is known, the Doppler equation outlined previously can be rearranged as:
V=cFd2F0cosθ
Types of Doppler Ultrasound
There are three types of Doppler ultrasound, namely continuous wave, pulsed wave, and color flow Doppler.
Continuous Wave Doppler.
In continuous wave Doppler, one piezoelectric crystal is used for transmitting a continuous wave at a fixed frequency, and a second crystal is used continuously to record the reflected signals. Both crystals are embedded within the same transducer with a slight angle toward each other, and the Doppler shift is continuously sampled. As an ultrasonic wave is transmitted continuously, no spatial information is obtained. Indeed, all velocities occurring anywhere within the ultrasound beam, in other words on the selected ultrasound line of interrogation, will contribute to the reflected signal and will appear in the spectrogram. As the ultrasound signal weakens with depth due to attenuation, velocities close to the transducer will intrinsically contribute more than the ones occurring further away. The major advantage of continuous wave Doppler is its ability accurately to measure high frequency shifts with no upper limit. It can be used, therefore, to measure high-velocity jets. To optimize the direction of the echo beam within the direction of the flow, cross-sectional imaging and color Doppler can be combined with continuous wave Doppler from the same transducer, producing so-called duplex scanning. This gives a visual impression of being simultaneous but is achieved by rapid and automatic switching between Doppler and cross-sectional imaging. Adding color Doppler imaging helps optimally to align the beam of interrogation with the direction of the flow of blood.
Pulsed Wave Doppler.
Pulsed wave Doppler has been developed to give spatial information on the detected velocities. The technique is not based on the Doppler principle but provides an output in a spectral display that looks very similar to the way the continuous wave signal is represented. However, the Doppler shift itself is not measured by the system. When using the pulsed wave system, an image line is chosen along which ultrasonic pulses are transmitted at a constant rate. This rate is the pulse repetition frequency. Instead of continuously sampling the backscattered waves, only one sample of the reflected wave is taken at a fixed time after transmitting a certain pulse. This time interval is the range gate. The range gate will determine the exact depth where velocities are measured. The transducer sends pulses and must receive that signal back before other pulses can be transmitted. A sample volume is positioned at the area of interest. The velocity of sound in soft tissue is a given constant of 1540 m/s, and the go and return time is used to determine the depth of the sample volume. The velocities that can be measured are limited by the pulse repetition frequency, or the number of pulses that are emitted per second. Aliasing of the Doppler signal occurs when the pulse repetition frequency is too low and the returning signals from one waveform are not received before the next waveform is sent. The frequency at which aliasing occurs is also called the Nyquist limit. Aliasing will result in velocities being displayed at the same time below and above the baseline. The Nyquist limit will be lower the deeper the velocity is measured because the time for the wave to travel will be longer. The Nyquist limit depends on the frequency of the probe, with higher-frequency probes having lower Nyquist limits and lower-frequency probes having higher Nyquist limits. A 2.5-mHz transducer will display velocities of twice the magnitude of those produced by a 5.0-mHz transducer. Appropriate selection of the probe therefore is important when performing pulsed Doppler measurements. Different options are possible further to increase the Nyquist limits. The first is to shift the baseline so that velocities are measured in only one direction. A second method is to send out a new pulse before the previous one has returned. This is called the high-repetition frequency method and results in measuring velocities in more than one site or sample volume, thus reducing the spatial resolution but increasing the Nyquist limit. The size of the sample volume can also be adjusted. A smaller sample volume will result in a sharper velocity profile because fewer velocities are sampled at the same time.
Color Flow Doppler.
Color Doppler displays the direction and flow velocities of the blood superimposed on the cross-sectional image. In this technology, different pulses are sent across an image line, and the phase shift between the different signals is measured at two sampling points. This phase shift is proportional to the velocity of the reflecting object. A color is assigned to the direction of flow according to whether it is away from or toward the transducer. In this respect, it may be helpful to remember the mnemonic BART (“blue away and red toward”).
The velocity of flow is displayed in shades of these colors. The brighter the color, the higher the velocity. The color information represents the mean velocity of flow. When the flow is disturbed, or not laminar, the pattern will show as a mosaic. This mosaic pattern is produced only if the variance is on. The variance is a color Doppler option that is present with all the cardiac presets. It is important to appreciate that, because color flow mapping is a form of pulsed Doppler, it is subject to the same physical principles. Increasing velocities are represented as increasingly bright forms of red or blue until they reach the Nyquist limit, when aliasing then superimposes new colors on the display. Typical Nyquist velocity limits are from 0.6 to 1 m/s. Therefore for flows at high velocity, multiple aliasing occurs. The color image is then useful only from a qualitative point of view and does not permit the demonstration of directional flow. Similarly to pulsed Doppler, the Nyquist limit is dependent on transducer frequency and depth.
Color flow Doppler provides information regarding the Doppler shift from an entire area, unlike pulsed Doppler, which samples from a specific point. Therefore more time is required for color Doppler to compute the lines of information onto the screen. Frame rate and line densities are reduced proportional to the time required. Keeping the color sector small will provide a better frame rate and produce a flicker-free image. When using color flow, the operator is able to visualize the flow of blood in relation to the surrounding structures, which provides a method for rapid interpretation of abnormal location and direction of flow, and helps to guide Doppler interrogation of abnormal flow.
Comparison of Doppler Methods
The three types of Doppler interrogation described are complementary, each measuring the velocities of flow in different ways. For the evaluation of high velocities, the method of choice is continuous wave Doppler because it does not give rise to aliasing. Although continuous wave does not permit gating for precise localization of the target, a steerable cursor line and a focused beam allows precise alignment, thus assuring appropriate measurements of flow. Pulsed Doppler, in contrast, enables measurements of flow at a known depth, allowing more precise calculations, but is limited by the maximal measurable velocity. Color flow Doppler is a qualitative method but provides spatial information not obtained with other methods. By permitting visualization of the disturbed jet, it facilitates the alignment of the continuous wave Doppler beam. The visual effect of color flow Doppler provides a method of rapidly screening abnormal velocities within the heart, thus directing the more quantitative methods.
Image Optimization
M-Mode Imaging
M-mode echocardiography is derived from an M line superimposed on a cross-sectional image. The M-mode trace itself shows time as the second dimension. Control of the speed of the sweep enables accurate measurements of intervals in the cardiac cycle, and the high-repetition frequency of the technique allows not only excellent temporal resolution of moving structures but also precise measurements of mural thickness and cavity size. In this way, the information derived is superior to that obtained from B-mode imaging. M-mode echocardiography is still commonly used for the evaluation of left ventricular function, using short- or long-axis sections through the left ventricle, and the timing of cardiac events such as left ventricular ejection time, using a short-axis section through the aortic valve. Although it is no longer recommended by the most recent pediatric quantification guidelines, M-mode is still widely used in most pediatric laboratories and has advantages in young children with high heart rates because of the excellent temporal resolution.
Cross-Sectional Imaging
Although adults have the disadvantage of having poorer imaging windows often affecting penetration and signal-to-noise ratio, this rarely is a problem in younger children. Typically the imaging windows are better, resulting in higher-quality images. Lower depths allow the use of higher frequencies, therefore improving spatial resolution. Children have heart rates requiring a higher temporal resolution especially for visualizing rapidly moving structures like valve leaflets. Image optimization will always be a compromise between spatial and temporal resolution. A few general principles can result in image improvement:
- 1.
Always start scanning with the highest possible transducer frequency because this optimizes spatial resolution. For pediatric scanning, high-frequency probes (8 to 12 MHz) must be available and used. When penetration depth is limiting image acquisition, a lower frequency probe can be used. Typically, different transducers are used for different parts of the exam. For subcostal imaging, higher penetration is required and typically probes between 5 and 8 MHz are used depending on the child’s age and body size. For parasternal imaging, higher-frequency probes are used, typically in younger children and infants.
- 2.
In infants, scanning using fundamental frequencies generally provide good-quality images. Harmonic imaging should be avoided in younger children because it reduces axial resolution. If penetration and low signal-to-noise ratio becomes problematic in older children, using harmonic frequencies can improve image quality.
- 3.
Current machines provide good presets with automated image optimization for different patient sizes and ages. Optimal use of the presets will improve overall image quality. Optimizing two-dimensional images may require manually changing gain and dynamic range settings (compression) so that the structures of interest can be seen with the highest possible definition. time gain compensation is used to make the images as homogeneous as possible at different depths (particularly to reduce near field gain). Image depth and focus are always optimized to image the structures of interest.
- 4.
For optimizing temporal resolution, use the narrowest sector width where possible.
- 5.
Depth settings are minimized to include the region of interest.
Blood-Pool Doppler
Optimizing the Settings for Continuous Wave Doppler
The continuous wave image is an echocardiographic image that is influenced by all the parameters that affect a normal cross-sectional picture. The gain controls, therefore, should be manipulated to produce a clean uniform profile, without any blooming. Typically the Doppler gain should be increased not to miss any information, and the gain needs to be adjusted to reduce blooming of the signal that can result in overestimating velocities and gradients. The compress control assigns the varying amplitudes a certain shade of gray. If this control is either very low or very high, the quality of the spectral analysis graph will be affected, and this may lead to erroneous interpretation. The reject control eliminates the smaller amplitude signals that are below a certain threshold. This helps to provide a cleaner image and may make measurements more obvious. The filter is used to reduce the noise that occurs from reflectors produced from walls and other structures that are within the range of the ultrasonic beam. The volume button should be at the appropriate level to hear the frequencies.
Optimizing the Settings for Pulsed Doppler
The frequency of the probe will affect the Nyquist limit of pulsed Doppler, with probes of higher frequency having a lower Nyquist limit. The gain control, compress, and filter settings are similar to those described previously for continuous wave Doppler. Shift of the baseline allows the entire display to be used to show either forward or reverse flow, a feature that is useful if the flow is only in one direction. The scale should always be optimized and be set no higher than necessary to display the measured velocities. The size of the gate should be optimized, with an increase in the sample volume increasing the strength of the signal at the expense of a lower spatial resolution. In general, the smallest sample volume providing an adequate ratio of signal-to-noise should be used. The update function allows for simultaneous duplex imaging to optimize the location of sampling relative to the cross-sectional image. However, simultaneous cross-sectional imaging reduces the temporal resolution. All pulsed Doppler traces, therefore, should be obtained with the cross-sectional image frozen.
Optimizing the Settings for Color Doppler
This involves optimizing the gain settings, the scale, and the size of the sector. The gain should be adjusted until background noise is detected in the color image and then reducing it so that the noise just disappears. The scale should be adapted depending on the velocities of the flows measured. When examining high velocity flow, the scale should be adapted so the maximal Nyquist limit is chosen. When low velocity flow is studied, such as venous flow or flow in the coronary arteries, the scale needs to be lowered. The size of the color sector needs then to be adjusted to optimize frame rates. The smallest necessary size should be used.
Storage and Reporting of the Images Created
All current echocardiographic machines allow the recording and storing of images in a digital format, which can be retrieved, viewed, and further analyzed on a digital viewing system and workstation. This allows easy storage and retrieval of echocardiographic images and studies. More and more centers use digital systems for reporting, which can be integrated within the reviewing stations. The full digital workflow contributes to the quality and efficiency of pediatric echocardiographic laboratories.
Normal Cardiac Anatomy
When performing cross-sectional echocardiography, a thorough knowledge of normal and abnormal cardiac morphology is essential. In the normal subject, the long axis of the heart is oblique, extending more or less from the left subcostal region to the right shoulder ( Fig. 19.1 ). In addition to the long axis being oblique, the cardiac chambers, particularly the ventricles, are arranged so that the morphologically right structures are anterior to their morphologically left counterparts (see Chapter 2 ). The left atrium is the most posterior of the cardiac chambers. Only its appendage projects to the border of the cardiac silhouette. The right ventricle lies anterior to the left ventricle. It swings across the front of the ventricular mass, reaching from the inferior and right-sided tricuspid valve to the anterosuperiorly and leftward positioned pulmonary valve. The aortic valve is centrally located within the heart and is related to all four chambers ( Fig. 19.2 ). This central position of the aortic valve, located between the tricuspid and mitral valves, is the key to the understanding of cardiac anatomy. Because of the wedged position of the aortic valve, the subaortic outflow tract interposes between the leaflets of the mitral valve and the ventricular septum. Hence there are no direct attachments of the tension apparatus to the muscular septum in the left ventricle. In contrast, the septal leaflet of the tricuspid valve hugs the septum ( Fig. 19.3 ) and is attached to it by tension apparatus along its length. The short-axis sections emphasize other significant differences between the right and left sides of the normal heart. The arterial and atrioventricular valves in the right ventricle are separated by the supraventricular crest. No such muscular crest exists in the roof of the left ventricle, where the leaflets of the aortic and mitral valves are in fibrous continuity ( Fig. 19.4 ). The subaortic outflow tract also has important relationships to the areas of atrioventricular contiguity, these being made up of two components. The first, the atrioventricular membranous septum, is made of fibrous tissue, and is an integral part of the central fibrous body. It interposes between the medial wall of the subaortic outflow tract and the right atrium. The other area is the region of overlapping of the atrial and ventricular musculatures. It exists because the septal leaflet of the tricuspid valve is attached to the septum more toward the ventricular apex than are the leaflets of the mitral valve, producing the characteristic off-setting of the valvar leaflets. In the area between these valvar attachments, the walls of the right atrium overlap the base of the ventricular mass, thus forming a muscular atrioventricular sandwich ( Fig. 19.5 ). The anatomist describes the location of this area as seen from the diaphragmatic surface of the heart as the cardiac crux, representing the position where the plane of the normal septal structures crosses the inferior atrioventricular groove. The echocardiographer cannot see this point on the epicardial surface of the heart but is able to identify the so-called echocardiographic crux, the asymmetric cruciate appearance representing the off-set attachment of the atrioventricular valvar leaflets.





Anatomic Principles of Echocardiography
Echocardiographic imaging of the heart is obstructed by both bony structures and the air-filled lungs. Unobstructed views can generally be obtained from the cardiac apex, from alongside the sternum through the intercostal spaces, from beneath the rib cage, and from the suprasternal notch ( Fig. 19.6 ). Different factors can interfere with obtaining the standardized imaging views such as body size and lung interference. Especially in adult postoperative patients, windows can be very limited and additional imaging techniques may be required to obtain the information required. Transesophageal echocardiography allows visualization of the cardiac structures from the esophagus and stomach but is a more invasive technique that requires general anesthesia in children. Other imaging modalities like cardiac magnetic resonance imaging may be useful complementary techniques. A standard full echocardiographic protocol has been proposed for pediatric scanning combining different windows and views. (Lai et al.). A full pediatric study requires combining all windows and views to reconstruct the entire morphology and assess valve and ventricular function. Standard echocardiographic views are well established for patients with normally located hearts but can challenging in patients with abnormal position of the heart within the chest such as dextrocardia, with or without mirror-image atrial arrangement. This often requires using nonstandard positioning of the probe keeping the orientation of the probe so that the position of the heart within the chest is correctly displayed (especially left-right orientation). The position of cardiac structures in the chest is described using the patient’s coordinate system as a reference (patient’s left-right, superior-inferior, anterior-posterior). Orthogonal imaging planes are described relative to the body axis as coronal, sagittal, and transverse, but it should be mentioned that the axis of the heart rarely corresponds to the axis of the body (see Fig. 19.1 ). As the echocardiographer obtains the images based on the cardiac axis, it is more relevant to describe the images relative to the cardiac axis. Of the three cardiac orthogonal planes, two are in the long axis of the heart itself (parasternal long-axis and apical views), the other being in the cardiac short axis ( Fig. 19.7 ). Simple geometric principles dictate that, from any given window, it is possible to obtain only two of these basic planes, although intermediate cuts can be taken toward the third plane.


Scanning from the apical window provides the two cardiac long-axis planes. The coronal long-axis plane is at right angles to the inlet part of the ventricular septum and is conventionally termed the four-chamber plane (see Fig. 19.3 ). This is the plane that best shows the anatomy of the atrioventricular connections. When taken through the membranous atrioventricular septum, the section also incorporates part of the subaortic outflow tract. Hence most of these cuts show more than the four basic cardiac chambers. Nonetheless, the designation as a four-chamber plane is a useful one. The long-axis plane at right angles to the outlet part of the septum can, by analogy to the four-chamber plane, be considered as a two-chamber plane. In most cases it also includes part of the right ventricular infundibulum and illustrates more than the two basic chambers. The long-axis plane basically showing two chambers is also one of the standard planes obtained from the parasternal window ( Fig. 19.8 ), together with the series of short-axis planes ( Fig. 19.9 ). When scanning from subcostal and suprasternal windows, it is no longer possible to section a normally positioned heart in its own axes, although this may be possible when the heart is abnormally positioned. Instead, the usually located heart is sectioned in paracoronal and parasagittal planes relative to the axes of the body. From the subcostal position, such sections produce imaging planes that are similar to, but differ subtly from, the four-chamber and short-axis planes. These sections also highlight the location of the membranous septum and how the inferior part of the muscular ventricular septum separates the right ventricular inlet from the outlet of the left ventricle ( Fig. 19.10 ). These sections also show the long axis of the aortic arch, although this is probably better seen from the suprasternal window. Many views must be used to obtain a complete impression of cardiac structures. Despite the need for presentation and description of echocardiographic findings in terms of imaging planes, the key to successful evaluation is to obtain continuous scans from one view to another (sweeps). This allows the echocardiographer to build up a three-dimensional reconstruction from a continuous series of two-dimensional parts. By combining different sweeps, the entire cardiac anatomy can be imaged and reconstructed mentally.



Transthoracic Echocardiography
A full echocardiographic study involves a complete description of cardiac anatomy, valvar function, and cardiac systolic and diastolic function. Transthoracic windows are excellent in the majority of infants and children, so that interrogation from these windows can provide all anatomic, hemodynamic, and functional information required for diagnosis and treatment of most of the congenital and acquired cardiac lesions encountered by the pediatric echocardiographer. The majority of children are currently referred for cardiac surgery based only on transthoracic echocardiographic studies. However, for those aged between 3 months and 3 years, lack of cooperation can be a limiting factor, and sedation is generally required to permit performance of an adequate echocardiographic study. Transesophageal imaging in children is mostly limited to perioperative imaging.
The description of cardiac anatomy is best accomplished by using the segmental anatomic approach. By combining different echocardiographic windows, it is possible to recognize the precise anatomy of each cardiac segment, along with their interconnections and relations. This chapter concentrates on normal findings. The echocardiographic images of congenitally malformed hearts are described in other chapters. A detailed discussion of functional echocardiography is beyond the scope of this text; instead, our focus is mainly on the description of cardiac anatomy and valvar function.
When starting a full cross-sectional study in a new patient, most echocardiographers will prefer to start with subcostal imaging because this allows inferential determination of the arrangement of the abdominal organs and the heart. The study then proceeds with obtaining parasternal long-axis, short-axis, apical, and suprasternal views. A study performed for the purposes of follow-up will often start with parasternal long-axis views, deferring the subcostal imaging until the end of the examination. To help the echocardiographer to reconstruct the three-dimensional anatomy, two-dimensional sweeps can be recorded using the different cuts. For instance, a subcostal coronal sweep from posterior to anterior provides extra information in the third dimension. All images should be presented in their correct spatial, or attitudinally appropriate, position on the screen. The anterior and superior structures, therefore, are displayed at the top of the screen, and the rightward structures are generally placed on the left side of the display, with the exception of the parasternal long-axis cut when, by convention, the cardiac apex is displayed on the left of the screen. The standard views required for a pediatric echocardiographic study, as defined by the American Society of Echocardiography, are all obtained as part of a routine examination. These are the subcostal, apical, parasternal, suprasternal notch, and right parasternal cuts.
Subcostal Views
Different subcostal views can be obtained. Typical sections are obtained in a coronal plane, giving long-axis views, in the sagittal plane producing short-axis sections, and in the transverse plane. Subcostal images are presented in the anatomic orientation as the heart is positioned in the chest (apex down). Subcostal imaging begins with a transverse section to determine the arrangement of the abdominal organs by inference from the location of the abdominal aorta and inferior caval vein relative to the spine ( Fig. 19.11 ). The abdominal vessels can also be imaged in a long-axis view by rotating the probe 90 degrees counterclockwise. Color imaging will help to distinguish the identity of the abdominal great vessels. Posterior long-axis sections, with the transducer directed to the left of the midline, provide excellent views of the atrial septum ( Fig. 19.12 ). As the transducer is tilted anteriorly, the superior caval vein, the ventricles, and the left ventricular outflow tract can be imaged ( Fig. 19.13 ). Further anterior tilting of the probe provides views of the right ventricular outflow tract. A long-axis sweep from posterior to anterior is obtained. Counterclockwise rotation of the transducer, with the transduced notch positioned inferiorly at 6 o’clock, will produce different short-axis sections as the transducer is swept from right to left in a parasagittal plane. A section through the atriums allows imaging of the superior and inferior caval veins and the atrial septum ( Fig. 19.14 ). As the transducer is moved toward the left, the subcostal short-axis section of the left ventricle and the right ventricular outflow tract can be imaged ( Figs. 19.15 and 19.16 ). In this view, the right ventricular apex and outflow tract are seen, together with the pulmonary valve and proximal pulmonary trunk (see Fig. 19.15 ). By tilting the transducer further to the left, the midapical and apical portions of both ventricles can be imaged, along with the corresponding portions of the ventricular septum.
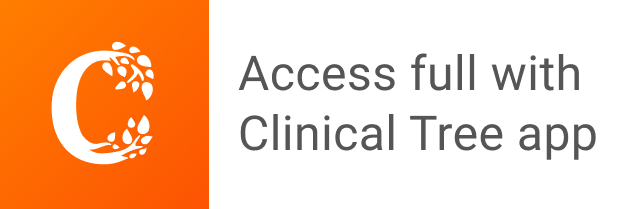