, Rohit Arora3, 4, Nicholas L. DePace5 and Aaron I. Vinik6
(1)
Autonomic Laboratory Department of Cardiology, Drexel University College of Medicine, Philadelphia, PA, USA
(2)
ANSAR Medical Technologies, Inc., Philadelphia, PA, USA
(3)
Department of Medicine, Captain James A. Lovell Federal Health Care Center, North Chicago, IL, USA
(4)
Department of Cardiology, The Chicago Medical School, North Chicago, IL, USA
(5)
Department of Cardiology, Hahnemann Hospital Drexel University College of Medicine, Philadelphia, PA, USA
(6)
Department of Medicine, Eastern Virginia Medical School Strelitz Diabetes Research Center, Norfolk, VA, USA
Distinct from chronic care, this chapter will cover several areas of critical care, including anesthesiology, the emergency room, the operating room, and the various intensive care units, highlighting the neonatology intensive care unit in the neonatology section. The chapter will close with a separate look at brain injury.
The P and S nervous systems control and coordinate the function of all of the visceral organs to promote homeostasis. These functions include heart and lung action, blood volume, BP, reactions to stress and fatigue, reactions to loss of oxygen profusion (e.g., to the brain), bleeding, hyperthermia and hypothermia, reactions to medical treatments and therapies and levels of anesthesia, and reactions to chemical and biological insults. There is a predictive nature to P&S monitoring, in that given a human’s basic desire to live, the P and S will work for a period of time to promote life once that human has received an insult. P and S post-insult activity deviations from the normal routine maintenance of homeostasis are detected by P&S monitoring. P&S monitoring may document these deviations earlier than any other indicators and therefore be an early biomarker of damage, distress, or disease. Thus, P&S monitoring has applications in routine health checks, wellness care, deployment monitoring, and critical and emergency healthcare.
Introduction
Critical care often carries the distinct disadvantage that the healthcare providers have no history on the patient prior to the need for therapy and intervention. P&S monitoring provides more information. Given that the ANS is primarily responsible for homeostasis, a harmony within the life of the patient, the balance between the P and S nervous systems reflects normal P and S function resulting in harmony, indicating normal homeostasis. Should homeostasis be upset due to damage, disease, or dysfunction, this harmony is broken and the balance is lost providing another, and sometimes earlier, indication of pathology [1]. There are some basics unique to critical care that require attention. Often in critical care, patients are mechanically ventilated. This raises concerns in P&S monitoring regarding the use of RSA to isolate parasympathetic activity. In free-breathing individuals, subtle changes in the respiratory cycles are also indicative of autonomic outflow and the influence of the P and S on the integrity pulmonary homeostasis. With mechanical ventilation, it seems as if the parasympathetics entrain to the rate set mechanically. Therefore, whether freely ventilated or mechanically ventilated, respiratory activity (RA) analysis provides information as to how the parasympathetics and the sympathetics are responding to and compensating for the RA that is present [1].
In critical care, often patients are unable to perform all aspects of the clinical autonomic study, including deep breathing (DB), Valsalva, or postural change (PC, e.g., stand). The 5 min baseline portion of the clinical study offers insight into morbidity and mortality risk and response to disease, disorder, or injury, and therapy. Resting P&S monitoring measures, P&S activity levels (RFa and LFa, respectively), and balance (sympathovagal balance, SB) provide information regarding pain levels, cardiovascular stress, risk of sudden cardiac death, tissue perfusion, and therapy titration, to name a few. The 5 min of baseline may be extended (virtually) indefinitely. Changes in P or S tone or SB reflect changes in therapy and patient physiology. In the OR or ER, continuous monitoring is recommended, providing immediate feedback on the patient’s physiology and response to intervention and therapy. In the ICU, continuous monitoring quickly becomes tedious. However, 5 min baseline recordings several times daily, every 4–6 h depending on the state of the patient, are clinically trendable, as if the patient were continuously monitored [2, 3].
The interrelationships between SB, hemodynamic changes, and cardiac arrhythmias are complex, and whether P and S activity is primarily responsible for hemodynamic disturbances or the occurrence of arrhythmias or an epiphenomenon may never be known. However, the P&S measures always reflect autonomic involvement [4–8]. P&S monitoring provides information independent from that of the perceived clinical severity of infarction, ejection fraction, sepsis, pain, shock, etc. Typically in the emergency department, patients are hydrated and medicated to normalize HR, BP, cardiac output, and blood oxygen levels. Yet even with these efforts, morbidity and mortality risk remains high. Granted this may be the nature of the setting, but by the time the hemodynamic parameters change sufficiently to drive therapy, often there is little time for healthcare providers to react. P&S monitoring provides more information earlier and enables physicians to be more proactive.
Part of the dilemma is that HR, BP, and cardiac output are assumed to be sympathetic function, when in point of fact they are the result of the activity of both the P and the S branches of the autonomic nervous system. Perhaps when the patient first presents in the emergency department, with high HR and BP, the assumption that they are mostly sympathetically driven is valid. However, as soon as therapy is initiated, this assumption is no longer valid, for the purposes of therapy (i.e., beta-blockers, specifically known as beta-1 adrenergic antagonists, as one example) are to reduce sympathetic activity. Once sympathetic activity is reduced, the above assumption is no longer valid, and often patients persist in their morbidity or mortality even though their HR, BP, or cardiac output is normalized. Independent, simultaneous parasympathetic measures eliminate the need for assumption by providing more information. Less well known is the association between parasympathetic activity and tissue perfusion (as measured by PtCO2/FIO2, transcutaneous oxygen indexed to fractional inspired oxygen) and blood oxygenation (SpO2). PtCO2/FIO2 and SpO2 are both highly negatively correlated with parasympathetic activity [2].
The last general point to consider is autonomic neuropathy. As all who have worked with patients diagnosed with diabetes in the critical care setting have experiences, patients with diabetes pose an additional risk because of the known earlier onset of autonomic neuropathy. Diabetic autonomic neuropathy (DAN) and cardiovascular autonomic neuropathy (CAN) are conditions that are “need to know” for these patients. CAN is the result of very low autonomic tone, specifically defined as resting parasympathetic activity (RFa) less than 0.1 bmp2. CAN is associated with compromised cardiac and vascular control. CAN indicates risk for sudden cardiac death (SCD) and abnormal responses to anesthesia and other critical care interventions. The risk for SCD is stratified by resting autonomic balance (SB). The more abnormal SB is, the higher the mortality risk. DAN is the precursor for CAN in diabetics. DAN is defined as either P or S activity less than 0.5 bpm2. DAN with anesthesia or other forms of intervention may quickly become CAN and then escalate the mortality risk, and this may happen silently (asymptomatically). Please be aware that DAN is not the province of diabetics alone. DAN’s correlate in nondiabetics is known as advanced autonomic dysfunction and in nondiabetics carries the same weight as does DAN in diabetics [9, 10].
Anesthesiology
Decreased HRV in surgical patients is associated with intraoperative hypotension and bradycardia [11], particularly in diabetics [12–16]. Amour and Kersten [17] noted that CAN significantly contributes to the pathogenesis of diabetic cardiomyopathy, with an associated increased perioperative morbidity and mortality. Left ventricular dysfunction in diabetic patients is related to CAN [18, 19] with its nonuniform loss of cardiac neurons causing a sympathetic imbalance [20]. Valensi et al. [21] reported that in diabetics, CAN is a stronger predictor for a major adverse cardiac event (MACE) than silent MI. In addition to the arrhythmias associated with CAN, patients with CAN experience greater platelet activation compared to patients without CAN, contributing to a MACE [22]. Perioperative P&S monitoring elucidates P and S activity changes that may be useful in maintaining the patient [23 , 24]. A very strong relationship has been demonstrated between the severity of diabetic retinopathy and CAN in NIDDM patients [25], in turn correlating with reduced survival [26, 27]. Hemodynamic instability during vitrectomy surgery is related to preoperative ANS dysfunction [14]. The highest mortality rates associated with diabetes occur in those patients with nephropathy [28, 29] and CAN [30–33]. Long-term survival after surgery for diabetic retinopathy is decreased by renal disease [30, 34], including proteinuria [26, 28, 35, 36]. In diabetic dialysis patients, CAN is strongly associated with left ventricular hypertrophy and believed to be a key factor in the high prevalence of MACE in these patients [37].
Aging is accompanied by autonomic dysfunction [38–44]. It has been said that with aging all persons will develop CAN. Calculations for ANS staging are based on tables according to age [45]. As African Americans are well known to have a higher prevalence of diabetes [46], it might be expected that the prevalence of advanced autonomic dysfunction would be greater in African Americans. Parmer and colleagues [47] studied racial differences in HRV and found none. This may be due to the differences between HRV and P&S monitoring. Karter et al. [48] did observe increased end-stage renal disease in African Americans, even in those with insurance, but the other complications of cerebrovascular accident, CHF, or amputation were not increased in diabetic African Americans. The heart rate-correlated QT interval (QTc) is considered an indicator of imbalanced cardiac ANS activity, predisposing to arrhythmias, including ventricular fibrillation [33, 49], and to death [50, 51]. An abnormal QTc is defined as a QTc > 440 for females and >430 for males [51]. Multiple studies have found a significant correlation between the QTc, CAN, and SCD [51–55]. The combination of both a prolonged QTc and CAN is strongly associated with long-term mortality in diabetics with albuminuria, independent of other risk factors [56, 57]. Albuminuria, an indicator of endothelial dysfunction and vascular damage in diabetes, is associated with increased long-term mortality [28, 58]. Long-term survival after surgery for diabetic retinopathy is decreased by renal disease [34], including proteinuria [26, 28]. Boyd et al. presented data [9, 10] which are consistent with those of Lacigova et al. [59], wherein asymptomatic diabetics with albuminuria, peripheral neuropathy, and hypertension presenting to outpatient clinics were found to have an 85 % prevalence of CAN.
Beta-blockers improve HRV [60–62] and prognosis [63] in CHF patients and after an MI [64]. Angiotensin-converting enzyme inhibitor increases total HRV improving SB in patients with mild, but not advanced, autonomic neuropathy [65]. The addition of an angiotensin receptor blocker further improves hemodynamics and neurohumoral control [65, 66]. Burgos et al. [13] excluded all patients taking these medications, while all patients in the Hanss [11] study received medications for hypertension, arrhythmia, or CHF. Multiple investigators have recommended preoperative screening for autonomic dysfunction in diabetics [13, 67] and in the overall surgical population [11, 12, 68, 69]. Indicative of the importance of measuring autonomic function, clinically apparent ANS symptoms like orthostatic hypotension occur relatively late and do not correlate well with the severity of autonomic neuropathy [70]. Including assessment of ANS function in the preanesthetic evaluation may improve anesthetic risk stratification [15, 68, 71, 72]. Because cardiac [73–75] and neurodegenerative diseases, in the absence of diabetes, are associated with autonomic dysfunction [58], preoperative ANS testing could prove beneficial, perhaps leading to an objective predictive risk index. Unlike the American Society of Anesthesiologists status [76, 77], such a risk index would be reliably reproducible among different anesthesiologists. During anesthesia, diabetic patients with CAN are prone to bradycardia [16]. This holds for any with CAN. Knowing that advanced autonomic dysfunction may be present, the anesthesiologist can anticipate that anticholinergics may not be effective. Instead, the bradycardia in CAN patients is readily treatable with a small dose (20 mcg) of epinephrine.
Diabetic microvascular pathology can affect the entire brain [78, 79]. Bradycardia or hemodynamic instability should thus be immediately treated as diabetics appear not to tolerate episodes of reduced cerebral perfusion. The poor outcome of cardiopulmonary resuscitation in diabetic patients appears related to the cerebral microvasculopathy [80]. Hypotension during general anesthesia may result in poor outcomes [81, 82]. Given that general anesthesia can usually be avoided for retinal surgery, CAN-induced hemodynamic instability may be minimized [12, 15]. When a general anesthetic is needed, etomidate and sufentanil were found not to lead to hypotension in patients with ANS dysfunction [83]. During monitored anesthesia care for retinal surgery, propofol is frequently utilized, but is often associated with bradycardia, which has been demonstrated to be due to a decrease in the low-frequency sympathetic component of HRV [84, 85]. Continuous monitoring of ST segments (an indication of autonomic function) improves outcomes in advanced autonomic dysfunction patients with known history of silent ischemia and in whom radionuclide stress testing is neither predictive of a MACE nor sudden death [86–88].
At the 1990 Cleveland Clinic Symposium, Schubert presented a case for “Why is power spectral heart rate analysis of interest to the anesthesiologist” [89]. Hemodynamic responses to induction of anesthesia may be affected by patients’ cardiac disease, preoperative drug therapy, P and S activity, and anesthetic agents used. These hemodynamic alterations are difficult to predict, involve autonomic reflex mechanisms, and may produce myocardial ischemia during anesthesia by inducing bradycardia or hypovolemia. P&S monitoring quantifies physiologic state through measures of P&S activity [3]. It is well known that ANS conveys information to the sinus node in the heart through both its branches: efferent vagal and sympathetic pathways. The dissimilarities between the cholinergic (fast) and adrenergic (slow) speed of transmitter utilization account for differences in the modulation effects of these two systems on cardiac rhythm. Opioids tend to have a negative chronotropic effect, which seems to be more apparent when the drugs are administered rapidly or in large doses [90].
Cardiac stress testing has not been predictive of sudden death in diabetic patients, whereas CAN has been shown to be predictive [91]. Various clinical conditions have been reported to have a close association with CAN including diabetes, creatinine >2 mg/dl, and QTc > 430 ms. African Americans are known to have a higher incidence of diabetes and hence might be at greater risk for CAN [9]. Diabetics are known to be at risk for sudden death as well as hemodynamic instability during anesthesia, with a severalfold increase in perioperative morbidity and mortality compared to nondiabetics [92]. These adverse events are believed to be related to their autonomic neuropathy. With diabetes as a model of chronic disease, this may translate to an equivalent risk in nondiabetics with advanced autonomic dysfunction, the nondiabetic correlate to DAN. While radionuclide stress testing has been demonstrated not to be predictive of either sudden death or a major cardiac event, cardiac autonomic dysfunction has been shown to be a strong predictor of ischemia [91]. Preoperative quantification of the prevalence and severity of the autonomic dysfunction may predict perioperative risk and aid in anesthetic management [10].
Bi-spectral Index (BIS) and other technologies used to measure depth of anesthesia are augmented by P&S monitoring. BIS, for example, measures cerebral or cortical activity to determine depth of anesthesia. This determines the patient’s level of consciousness and awareness of the activities during the operation. P&S monitoring measures brainstem activity and core or autonomic functions of the body that sustain life. Since anesthesia suppresses brainstem or autonomic function as well, if the patient’s autonomic function is already suppressed (e.g., due to DAN, advanced autonomic dysfunction, or CAN), a normal amount of anesthesia may be too much. P&S monitoring provides more information to aid the anesthesiologist. For longer surgeries, P&S monitoring helps to indicate when a patient may be coming light and helps the anesthesiologist to titrate agents to maintain sedation.
Three studies are available using P&S monitoring in anesthesiology [3, 9, 10, 90]. Their summaries are presented here.
Extensive changes in hemodynamics and cardiac rhythm during induction of anesthesia may be mediated by altered P and S responses to anesthetic agents. P&S monitoring supplies more information about the ANS and may be used to assess this phenomenon. In this study, published in 1992 [90], 78 patients undergoing coronary artery bypass graft surgery were evaluated. Anesthesia was induced with sufentanil and neuromuscular blockade with vecuronium, a combination that may cause a decrease in HR. Before and after induction of anesthesia, the HR, BP, cardiac output, cardiac index, and P&S responses were recorded. Alterations in HR after induction of anesthesia were defined in order to separate the patient population into two groups: slow HR and stable HR. Slow HR was defined as a decrease in HR of more than 20 % of the baseline value. The variables were analyzed and compared between the slow-HR (n = 25) and stable-HR (n = 53) groups in order to verify the possibility of identifying patients prone to hemodynamic changes after anesthesia induction. There were no differences in preoperative HR, BP, cardiac output, or cardiac index between groups before anesthesia induction. However, P&S monitoring showed a higher SB in the slow-HR (average SB = 7.30 ± 1.31) than in the stable-HR (average SB = 4.38 ± 0.63) population (p < 0.001). Sympathetic activity was not significantly different between groups. Parasympathetic activity in the slow-HR group (0.34 ± 0.08 bpm2) was lower than in the stable-HR group (1.04 ± 0.22 bpm2) before induction of anesthesia (p < 0.001). Even though the SB data suggests a greater SE in patients with high SB, these data showed that patients who developed a slow HR following induction of anesthesia had similar sympathetic activity and lower parasympathetic activity than patients who maintained a stable HR. (Relatively lower parasympathetic activity with comparable sympathetic activity will result in higher SB, just as relatively higher sympathetics with comparable parasympathetics.) Lower parasympathetic activity may identify patients at high risk of developing hemodynamic alterations following anesthesia. The present findings suggest that a low preinduction parasympathetic activity (the definition of CAN) may be associated with increased risk of a post-induction inadequate hemodynamic response [90]. Diabetics are known to be at risk for sudden death as well as hemodynamic instability during anesthesia, with a severalfold increase in perioperative morbidity and mortality compared to nondiabetics [92]. Again, these adverse events are believed to be related to their autonomic neuropathy [91]. Thus, preoperative quantification of the prevalence and severity of autonomic dysfunction may predict perioperative risk and aid in anesthetic management.
For the next study, there are two parts which were presented to the American Society of Anesthesiologists, Annual Meeting, 2010, a prevalence and severity scoring presentation [10] and a predictor presentation [9].
On the day of surgery, diabetic and nondiabetic patients underwent a standard Autonomic Assessment clinical study with the P&S monitor. See Chap. 5 of this compendium for a complete methodology describing the Autonomic Assessment study. The Autonomic Assessment section (Fig. 3.8) includes a copy of the original figure presented in this manuscript, depicting a model of the P&S monitoring method. Stages of autonomic dysfunction are defined as (0) none, (1) parasympathetic withdrawal (PW, an abnormally low parasympathetic response to DB challenge), (2) sympathetic release (an abnormally high sympathetic response to Valsalva challenge with normal PC (stand) challenge and resting responses), (3) sympathetic decline (an abnormally low sympathetic response to Valsalva challenge with normal PC challenge and resting responses), (4) sympathetic withdrawal (SW, an abnormally low sympathetic response to PC) with orthostatic dysfunction and decreased quality of life symptoms, (5) diabetic autonomic neuropathy (DAN, or advanced autonomic dysfunction defined as resting P or S activity less than 0.5 bpm2, and the resting parasympathetic activity greater than 0.1 bpm2), and (6) CAN (defined as resting parasympathetic activity less than 0.1 bpm2). The diabetic study subjects exhibited significantly more overall autonomic dysfunction (see Table 29.1) and were significantly more likely to exhibit CAN (p = 0.008) than the nondiabetic patients. Of the diabetic patients, only 1.9 % did not demonstrate ANS dysfunction, and 20.5 % had CAN with indication for risk of sudden death. CAN is associated with hemodynamic instability under general anesthesia. In patients with CAN, atropine may not be effective and epinephrine should be immediately available for treatment of sudden bradycardia and hypotension. A significant number of diabetic patients may be at risk for sudden death, major adverse cardiac events (MACE), and hemodynamic instability during retinal surgery. Preoperative Autonomic Assessment may help identify these patients. Preoperative P&S monitoring may become part of preoperative assessment of diabetic patients [10].
Table 29.1
Severity of autonomic dysfunction in diabetic and nondiabetic preoperative cases
Stage (N) | 0 | 1 | 2 | 3 | 4 | 5 | 6 |
---|---|---|---|---|---|---|---|
Diabetics (44) | 1 (2 %) | 0 | 0 | 3 (6 %) | 6 (14 %) | 25 (57 %) | 9 (21 %) |
Nondiabetics (52) | 6 (12 %) | 2 (4 %) | 0 | 7 (14 %) | 13 (25 %) | 23 (44 %) | 1 (2 %) |
p value | <0.008a |
From the predictor analysis, an ordinal regression model was fitted for the six stages of autonomic dysfunction and a logistic regression model for the yes/no presence of CAN. Both regression analyses used the four predictor variables of (1) race, (2) proliferative diabetic retinopathy compared to non-proliferative diabetic retinopathy, (3) creatinine >2, and (4) QTc > 430; p < 0.05 was considered significant. The results indicate that neither race, proliferative diabetic retinopathy, creatinine >2, nor QTc > 430 was significant predictors of autonomic dysfunction or specifically CAN as seen in Table 29.2.
Table 29.2
Clinical predictors of cardiac autonomic neuropathy in retinal surgery patients
Variable | Diabetics (N)/nondiabetics (N) | Positive for CAN | o value |
---|---|---|---|
Creatinine>2 | 10/0 | 3 | 0.999 |
PDR | 33 with PDR 12 with NPDR (all diabetics) | 8 | 0.131 |
AA | 22/5 | 3 | 0.861 |
QTc>430 | 20/9 | 3 | 0.538 |
Several clinical indicators have been suggested to predict CAN; none of these were found to be significant in this retinal surgery patient population. Preoperative measurement of the severity of autonomic dysfunction may thus allow better risk stratification than suggested clinical indicators and risk indices [91]. As CAN is known to result in hemodynamic instability during general anesthesia [93], knowledge of its presence aids in anesthetic management (e.g., atropine vs. epinephrine for sudden bradycardia or hypotension associated with CAN). The studied clinical predictors will not substitute for actual measurement of autonomic dysfunction. None of the clinical predictors of CAN proved to be significant in this retinal surgery population. Preoperative P&S monitoring may be valuable in risk assessment and anesthetic planning.
The third study was sponsored by the US Navy, ultimately to study the autonomic effects of blood loss in trauma. This study (1998–2002) considers a variety of patient anesthesia and surgical intervention effects as measured by P&S monitoring and hemodynamic techniques. Surgeries included elective laparoscopic cholecystectomy and emergency radical surgeries. Head trauma, either open or closed, was excluded. Data were collected during the immediate intraoperative period (while in the operating room): (1) preop ((a) immediate preoperative activities (including preoperative motion artifact), (b) anesthesia induction, (c) intubation, and (d) baseline), (2) intraop ((e) incision, (f) bleeding, (g) surgical stimulation, (h) intraoperative activities, (i) additional sedation, (j) intraoperative stable, (k) closing, and (l) extubation), and postop ((m) immediate postoperative activities and (n) return to consciousness).
P&S monitoring data collected from continuous baseline monitoring include (1) sympathetic activity (LFa in bpm2), (2) parasympathetic activity (RFa in bpm2), and (3) SB (unitless). See “Autonomic Assessment”, Chap. 5 for an explanation of the derivation of these parameters. The hemodynamic data collected include (1) HR in bpm, (2) mean arterial pressure (MAP, in mmHg), (3) arterial hemoglobin oxygen saturation by pulse oximetry (SapO2, %), and (4) inspired fraction of oxygen concentration (FIO2, unitless). Hemodynamic data were collected every 15 min throughout the period of P&S monitoring.
Patient Cohort
Fifty-seven (57) consecutively monitored laparoscopic cholecystectomy patients and radical surgery patients (44.88 ± 12.08 years) were studied: 26 were laparoscopic cholecystectomy patients (21 females, 37.0 ± 10.5 years), and 31 were radical surgery patients (10 females, 51.4 ± 9.2 years). Of the radical surgeries, 20 were radical prostatectomy patients, 2 were radical hysterectomy patients, 4 were exploratory laparotomy patients, and 5 had other radical surgeries (nontraumatic).
Results and Discussion
All of the data combined are presented in the Fig. 29.1.


Fig. 29.1
P&S monitoring and hemodynamic data from all patients for each operation phase studied. The events on the abscissa are in chronological order from left to right. The first four events (preoperative motion artifact, anesthesia induction, intubation, and baseline) are from the preoperative period. The intraoperative period is composed of those events from incision through extubation. The postoperative period includes the last two: immediate postoperative period and return to consciousness period
Preoperative Period
In the preoperative control period, there are appreciable increases in P&S activity (abbreviated in Fig. 29.1 as “RFa” and “LFa,” respectively), while MAP, HR, SapO2, and FIO2 are at their normal expected levels (see Fig. 29.1). Upon anesthesia induction P&S levels are normal (5.11 ± 4.26 and 3.67 ± 2.93, respectively) and reduced at the preoperative baseline (1.23 ± 0.36 and 1.63 ± 0.56, respectively). SB (abbreviated in Fig. 29.1 as “Ratio”) for the two time periods is normal (1.49 ± 0.63) and high (4.38 ± 1.47), respectively. P&S activity at induction shows large standard deviations. This may be due to the differing absorption rates of anesthesia since induction. The lower values for the P&S activity at the preoperative baseline show much smaller standard deviations; therefore, they are more reflective of the anesthetized state. SB at induction is in the normal range and increases after induction, indicating a relative sympathetic excess. This is probably due to the differential effects of the anesthesia on the individual autonomic branches.
The P&S activity is high on intubation (57.13 ± 17.93 and 31.08 ± 7.24, respectively), and SB is low normal (0.66 ± 0.08) indicating a relative parasympathetic dominance (see Fig. 29.1). During this time the MAP, HR, and SapO2 are essentially unchanged from their normal range. This is reflective of the stresses from the intubation still perceived at the brainstem level, even though the cortical perception is blocked by the anesthesia. The stresses, however, are not sympathetic; rather they are parasympathetic (since SB is low). Thus, the intubation stresses seem to be associated with vagal stimulation within the airways.
Intraoperative Period
The P&S values rise abruptly and strikingly at the time of the incision (105.48 ± 12.87 and 116.71 ± 16.33, respectively, see Fig. 29.1). SB is relatively unchanged from the preoperative baseline. Similar to intubation, the rise at the time of the incision seems to be the result of parasympathetic stimulation, again possibly due to vagal stimulation, this time through surgical manipulation of the peritoneum and viscera. The stability of SB indicates a relative stability in the SNS with respect to the PSNS, indicating the lack of (perceived) pain.
With the onset of bleeding, there are further increases in P&S activity, to the highest levels observed intraoperatively (161.51 ± 13.72 and 171.99 ± 14.02, respectively, see Fig. 29.1). Again, SB is relatively unchanged. The stimulation produced during surgical manipulation maintained the high levels of P&S activity (137.40 ± 13.52 and 137.33 ± 13.89) with SB relatively unchanged. These continue to point to a relative elevation in parasympathetic activity, possibly from (1) bleeding which causes a reduced venous return to the heart causing an increased parasympathetic response or (2) the viscera which is heavily innervated by the vagus nerve; thus, strong parasympathetic responses are reasonable upon surgical manipulation of the viscera. SB remains constant as a result of anesthesia maintenance and with a constant sympathetic response relative to parasympathetic activity, and there is no (apparent) perception of pain.
Although these patients were anesthetized, occasionally there is motion by the patient. The resulting P&S changes are less pronounced than for surgical manipulation (see Fig. 29.1: Intra-op Motion Artifact, indicated as point “A” on the abscissa). When additional doses of sedation are given (indicated as point “I” on the abscissa), there are pronounced reductions in P&S values. SB (“Ratio”) is appreciably increased (6.69 ± 4.44, abnormally high) exceeding previous levels during the addition of sedation, but returning to previous level when P&S stabilizes (2.29 ± 0.39, normal). The imbalance may be due to the drug effect or variation in time course of effect among the cohort, as confirmed by the high standard deviation. When the cohort eventually stabilizes, the standard deviation of SB is again within normal limits.
During the greater part of the operation while the patient could be described as stable, P&S activity is at its preoperative baseline range (post-induction). There are increases in P&S and SB activity during closing the operative wound. This, in part, may be attributable to the common practice of lightening the anesthetic dose 30–40 min prior to closing so that the patient would wake more easily postoperatively. The increase in SB indicates a relatively greater increase in sympathetic activity as compared with parasympathetic activity. This may be an early indication of perceived postoperative pain.
Postoperative Period
At the beginning of the postoperative period, patients are extubated. There are statistically significant increases in P&S activity upon extubation and reversal, and the patient is at least semiconscious (see Fig. 29.1). Like intubation, the increases in P&S activity seem to be reflective of parasympathetic stimulation through the Vagus Nerve. Relative sympathetic activity (i.e., SB) is lower than during the operation, possibly a function of the remaining anesthesia compounded by the opposing effects the parasympathetics have on the sympathetics.
In the immediate postoperative period, P&S activity (0.82 ± 0.25 and 0.62 ± 0.15, respectively) is higher by factors of almost 3 and 2, respectively, than during the intraoperative stable period indicating that the patient is returning to consciousness. At this time, SB (2.52 ± 0.47) has returned to normal (see Fig. 29.1), but is lower than the preoperative (post-induction) values, indicating stability and the possible absence of the preoperative anxiety. As the patient regains full consciousness, P&S activity increases significantly (103.30 ± 23.77 and 113.30 ± 40.02, respectively). The parasympathetic increase may be associated with the lower FIO2, indicating lower tissue oxygenation. The sympathetic increase may be due to pain. The fact that SB (1.87 ± 0.53) remains normal indicates that the opposing functions of the two ANS branches are balanced, suggesting that the patient remains stable.
Conclusion: Depth of Anesthesia
P&S monitoring detects and records in real time subtle brainstem responses to all stimuli. P&S monitoring provides a window into the depth of anesthesia as it relates P&S responses to the depressant effect of anesthetic agents. P&S monitoring records the amount of power in the P and S branches prior to anesthesia induction as the baseline for that patient at that specific time regardless of prior medicated or mental state. After anesthesia induction, the objective is to maintain enough power in the ANS to support life by titrating sufficient quantities of agent for that patient at that time. From Fig. 29.1, notice that both P&S activities decrease from “Intubation” to “Induction” and remain at the decreased levels for “Pre-Op Baseline,” as well as intraop baseline (“Added Sedation” and “Stable”). During reversal, the dosages may also be titrated more appropriately for that specific patient at that time and aid the physician in determining stability and appropriateness for postanesthesia care discharge. The data show significant and logical changes in P&S activity as correlated with the various surgical periods the patients’ experience.
Trauma
This next published study [4] investigates P&S activity and hemodynamic parameters in acute emergency patients with mild to moderately severe trauma. P&S monitoring of 14 mild to moderately severely injured patients (2 females, mean age 34 years) occurred within the first 1–4 h after admission to the emergency department (ED), before and during the initial fluid resuscitation, before radiological diagnostic studies, and before the use of sedation, anesthesia, or vasoactive agents. The mean ± standard deviation injury severity score was 10.1 ± 4.8. The patients were not under the effect of anesthesia; in three patients, mild sedation or one dose of pain medication was given. Fluid therapies were limited to intravenous infusions of crystalloids of not more than 100–500 mL prior to P&S monitoring. All study patients were discharged alive and well after 2–23 days of hospitalization. Patients were selected based on the following criteria: hypotension (systolic BP <100 mmHg or mean arterial pressure <70 mmHg), tachycardia (HR >100 bpm), multiple long bone fractures, head injuries, or blunt abdominal and thoracic injuries. Exclusion criteria included arrhythmias, seizures, shivering, and delays in onset of hemodynamic monitoring.
P&S monitoring and hemodynamic monitoring were performed on these patients, including bioimpedance cardiac output, HR, and mean arterial pressure (MAP) to measure cardiac function and transcutaneous oxygen (PtcO2/FiO2) to reflect tissue perfusion. P&S and hemodynamic activity from baseline through surges were examined. Surges were defined as values >25 units. The significance of the changes from baseline to peak and from peak to subsequent lowest level of each parameter was tested using the paired Student T-test.
Results
Over 31 h of continuous data were studied, and the P&S responses were compared with concomitant changes in the hemodynamic responses, including cardiac index, HR, MAP, and transcutaneous oxygen tension. Figure 29.2 illustrates continuous simultaneous patterns of HR (instantaneous HR or IHR) with P&S activity, upper and lower graphs, respectively. In 66 % of the data, increases in parasympathetic activity (RFa, upper graph) are associated with increases in HR, and decreases are associated with decreases. Similarly, in 89 % of the data, increases in sympathetic activity (LFa, lower graph) are associated with increases in HR, and decreases are associated with decreases. SB has the lowest (61 %, not shown) directional patterns with HR. These examples are repeated for cardiac index, stroke index, mean arterial pressure, and transcutaneous oxygen tension (not shown). Inspection of the continuous measurements reveals the presence of sudden bursts or surges of autonomic activity of widely varying magnitude that occur along with parallel changes in hemodynamic responses.


Fig. 29.2
Changes in instantaneous HR (IHR, upper curves in the two plots) and changes in parasympathetic activity (RFa, lower curves in upper graph) and sympathetic activity (LFa, lower curves in lower graph) plotted with changes in six representative surges in P or S activity (Adapted with permission from Fathizadeh et al. [4])
P&S activity increases in surges, defined by abrupt increases >25 bpm2. HR, cardiac index (CI), and mean arterial pressure (MAP) also demonstrate significant increases from baseline with sudden P&S surges (see Table 29.3). Tissue perfusion reflected by transcutaneous oxygen tensions (PtcO2/FiO2) significantly decreases from baseline during the surges of increases P&S activity. The sensitivity of HR patterns to P&S changes is not affected by the range of baseline HR values. There are pronounced changes in HR with surges of P&S activity at the baseline range to tachycardia and higher (see Fig. 29.2). With significant decreasing surges in sympathetic activity, the hemodynamic responses significantly decreased in response (see Table 29.4).
Table 29.3
Changes in hemodynamic values with increasing sympathetic surges
Baseline mean ± SD | Peak LFa mean ± SD | Paired-T p value | |
---|---|---|---|
LFa (bpm2) | 1.6 ± 1.57 | 28.9 ± 41.7 | 0.0093 |
RFa (bpm2) | 0.58 ± 0.73 | 15.2 ± 23.1 | 0.0097 |
SB | 11.4 ± 12.7 | 9.1 ± 15.4 | 0.280 |
CI (L/min/m2) | 2.94 ± 0.52 | 3.67 ± 0.89 | 0.0001 |
SI | 37.2 ± 10.2 | 39.9 ± 12.9 | 0.180 |
HR (bpm) | 84.9 ± 18.8 | 96.7 ± 21.5 | 0.0001 |
MAP (mmHg) | 91.2 ± 9.0 | 95.4 ± 9.7 | 0.0006 |
PtcO2/FiO2 | 333 ± 129 | 311 ± 142 | 0.040 |
Table 29.4
Changes in hemodynamic values with decreasing sympathetic surges
Baseline mean ± SD | Peak LFa mean ± SD | Paired-T p value | |
---|---|---|---|
LFa (bpm2) | 22.6 ± 33.7 | 1.31 ± 1.11 | 0.018 |
RFa (bpm2) | 19.8 ± 36.3 | 4.9 ± 12.8 | 0.150 |
SB | 8.9 ± 16.7 | 9.1 ± 11.4 | 0.970 |
CI (L/min/m2) | 3.7 ± 1.0 | 2.96 ± 0.57 | 0.0005 |
SI | 36.4 ± 15.0 | 33.7 ± 10.7 | 0.390 |
HR (bpm) | 99.7 ± 20.6 | 87.0 ± 16.1 | 0.0001 |
MAP (mmHg) | 96.4 ± 9.0 | 89.3 ± 10.1 | 0.0001 |
PtcO2/FiO2 | 320 ± 136 | 339 ± 166 | 0.450 |
Conclusions
Clinically, P&S monitoring documents P and S activity and whether the level of treatment is adequate for the individual patient at that time, given the patient’s history. Goals may be to titrate interventions to the level that is most effective at that given time. P&S monitoring has been used in studies of various health conditions such as sepsis [94], trauma and shock [1], depth of anesthesia [95–97], cardiac dysfunction [97–100], cardiopulmonary diseases [100], diabetic neuropathy [101–103], pain management [104], neonatal development [105–108], pharmaceutical interactions [109], and brain death [1, 110]. In these prior studies, patients have been monitored to compare the average P&S and hemodynamic values with values of normal populations.
Invasive monitoring has been the most definitive means of evaluating circulatory function in high-risk patients, but it is costly, is personnel intensive, has complications, and is often started late in the course of illness after ICU admission and after the onset of organ failure. Delays in management of trauma patients have led to circulatory deficiencies, organ failures, and death. However, P&S monitoring may reduce the risk and cost of monitoring by invasive techniques [1, 111–113]. New noninvasive methodologies may also reduce delays in instituting therapy and thereby improve outcomes.
Depicted in Fig. 29.3 are a series of surges in IHR and sympathetic (LFa) activity taken from coincident time periods. The solid saturation curve (“S-curve”) and upper (heavier) response curves are IHR data, and the broken S-curve and lower (lighter) response curves are sympathetic measures. It was found that increased P or S activity always precedes increases in all other hemodynamic activity measured. There are two pieces of evidence that demonstrate the fact that P&S activity precedes hemodynamic activity. First, the inflection point (the middle of the vertical portion of the S-curve) that marks the beginning of an increase is earlier for autonomic activity than for hemodynamic activity. In the example presented in Fig. 29.3, the vertical section of the sympathetic (LFa, broken line) S-curve is steeper than that for IHR. As a result the inflection point for this patient’s sympathetic activity occurs near the beginning of the vertical section of the IHR S-curve and well before its inflection point. Second, the top of the S-curve, indicating maximum response, is reached first by autonomic activity and then by hemodynamic activity. This is consistent with the concept that the P&S activity initiates changes in the hemodynamic parameters.


Fig. 29.3
A saturation curve, quiescent-point model of P&S and hemodynamic activity similar to those that describe neural function elsewhere in the human body (Adapted with permission from Fathizadeh et al. [4])
A Model of Autonomic Function
The graphs in Fig. 29.2 describing the relationship between P&S and hemodynamic data are reminiscent of saturation curves (S-curves) common in sensory neurophysiology. The nervous system, especially the sensory nervous system, has many examples of S-curve models fitting the data that describes sensory–neural function. The activity of hair cells and auditory encoding on the auditory nerve is a prime example. The data depicting the IHR and sympathetic data are represented in Fig. 29.3 with a typical S-curve overlaid to exemplify the point. These data lend themselves to an S-curve, quiescent-point model of P&S, and hemodynamic activity similar to those that seem to adequately describe neural function elsewhere in the human body. The model is depicted in Fig. 29.4. The ordinate indicates sympathetic activity level or power (in bpm2). The abscissa indicates the functional range of a parameter of interest, such as HR. Each condition, listed across the top of the figure (e.g., “resting” or “brisk exercise”), has a family of S-curves associated with it in the graph below it. Each S-curve is characterized by an operational range. The operating range is the steep portion of the S-curve. The two saturation regions are where the S-curve becomes flat (asymptotic). The midpoint of the S-curve (where they happen to intersect in this example) is the inflection point and for these types of models also known as the quiescent point (Q-point). The slopes define how fast the function switches from low to high and back or how sensitive the function is to small changes over a larger range. One extreme would be a step function (a square wave rather than a smooth “S”-curve); instantly “on” and instantly “off” (an infinite slope) and the other extreme would tend towards a horizontal line of nearly zero slope.


Fig. 29.4
The ordinate indicates sympathetic (SNS) activity level or power (in bpm2). The abscissa indicates the functional range of a parameter of interest, such as HR. Each condition, listed across the top of the figure (e.g., “resting” or “brisk exercise”), has a family of saturation curves (S-curves) associated with it in the graph below it. Each S-curve is characterized by an operational range that seems to be established by parasympathetic activity. The operating range is the step portion of the S-curve. The two saturation regions are where the S-curve becomes flat (asymptotic). The midpoint of the S-curve (where they happen to intersect in this example) is the quiescent point (Q-point). The slopes define how fast the function switches from low to high and back or how sensitive the function is to small changes over a larger range
From the theory and our data [94, 114–116], it seems as if the activity of the PSNS sets the Q-point and selects the particular S-curve depending on the current (general) level of activity. In the sensory nervous system, the Q-point establishes the sensitivity of the stimulus response, given stimulus conditions at the time. The fact that the shape and position of the S-curves change to match the stimulus conditions is also common. This ability to move enables the sensory epithelium to be sensitive to a very large range of stimuli, while conserving energy and resources. The parasympathetics are known to establish the metabolic threshold for a given condition, around which the sympathetics react to a specific stimulus. For example, if while you are sitting, relaxed, and you hear someone tell a joke which makes you laugh, you need only a small sympathetic surge to raise HR and BP a little to support the laughter. In this case, the parasympathetic level does not need to change, and it is not like you plan to run away (hopefully). The Q-point establishes the metabolic threshold requirements. The sympathetics react (surge) to change hemodynamic function (HR, BP, etc.) to move along a particular S-curve with a slope that best defines the instantaneous metabolic needs of the individual at that time.
As evidenced by the data for trauma patients, it seems as if the trauma set point is highly shifted to the right (comparable to brisk exercise) as compared to that for more normal activities. Of course, if the sympathetics continued to the right along one of the left hand S-curves (e.g., along of one of the resting S-curve’s upper asymptotes), it could eventually cause an HR or BP as high as if the parasympathetics shifted it there. However (without a change in parasympathetic activity), the sympathetically driven changes would require much more energy and time to reach the desired hemodynamic levels. Thus, it seems more likely that the parasympathetics are driving the whole curve there, enabling the sympathetics to maintain their sensitivity and specificity. This may be the reason for the difference between the level of correlation between parasympathetic surges and hemodynamic data (66 % [4]) and sympathetic surges and hemodynamic data (89 % [4]). Factors that can influence the slope of the S-curves include genetics, history, drugs, training, exposure, mood, hormones, environment, disease, and lifestyle.
Of course these are gross generalizations. The closed-loop feedback relationship between the ANS branches significantly blurs the separation of duty, but based on currently accepted thinking, this model seems to fit. It also seems as if this model is sufficiently flexible (or adaptable) to incorporate many of the subtle interactions within the ANS, especially as it becomes more apparent that the “duties” are truly shared rather than separated. For example, instead of a two-dimensional model, a three-dimensional (or higher) model is required. In other words, instead of only the parasympathetics shifting the saturation curve horizontally only, both autonomic branches may shift the curve simultaneously (in this case the parasympathetics horizontally and the sympathetics vertically). This automatically suggests more situations that can be addressed by the autonomics using the same (relatively) simple method that is known to occur elsewhere in the nervous system.
Application
This Q-point model seems to provide a basis for common disorders in critical care. Cardiovascular events such as SCD, MI, cardiac ischemia, pulmonary embolism, and stroke may occur when parasympathetic levels are excessively low (e.g., CAN) and HR or BP becomes excessively high over periods of time. Under these conditions, the S-curve does not move (or does not move fast enough) to minimize metabolic expenditure, and the sympathetics are forced into an operating range (the upper saturation region) in which large changes in sympathetic activity are required to cause small changes in HR or BP (as modeled by the “flatness” of the saturation portion of the curve in this region). These small changes feedback and, without a timely shift in the parasympathetic level, initiate a feedforward condition driving more and more sympathetic activity which may be unrecoverable. In this region of the saturation curve the sensitivity of the nervous system is pathologically abnormal. The result of more and more sympathetic activity may then result in a cardiovascular event, including a MACE end point.
If the model for vascular events were inverted and the operating point were on the lower limb of the saturation curve, again, large changes in sympathetic activity (along the ordinate) are required to generate changes in HR or BP (along the abscissa) as modeled by the “flatness” of the saturation curve in this lower region. Again, the sensitivity of the nervous system is pathologically abnormal, keeping the HR or BP too low in this case, forcing more and more sympathetic activity to be expended in the face of bradycardia or hypotension and related disorders.
Sepsis
The following are excerpts from a manuscript submitted for publication after having been presented at the American College of Chest Physicians Annual Meetings, 2005 [116] and 2006 [117].
Introduction
P&S monitoring elucidates the role of the activity of both autonomic branches in the developing hemodynamic patterns in patients with severe sepsis. P&S involvement in HR and BP control is well known. P&S activities may also be affected by associated clinical conditions such as blood loss, dehydration, sedation, pain, therapy, degree of injury sustained, delays in correcting hypovolemia, and surgical repair of injuries [113, 118–120]. A study in mild trauma [4] demonstrated surges of autonomic activity that immediately preceded increases in HR, mean arterial pressure, cardiac index, and reduced tissue perfusion. A study in septic patients [115] showed improved outcomes with goal-directed therapy that started in the emergency department based on noninvasive or minimally invasive monitoring.
The present study (a) describes P&S patterns during the early course of acute sepsis, (b) relates the P&S patterns to the evolving temporal hemodynamic patterns, (c) describes the P&S and hemodynamic interactions of sepsis both as a primary disease and as a complication of trauma and surgery, and (d) suggests a regulatory role of autonomic surges in the developing hemodynamic patterns of acute sepsis. Simultaneous noninvasive hemodynamic and P&S monitoring provide a unique opportunity to study the role of P&S activity in the hemodynamic responses to various clinical septic conditions alone and in combination with trauma or surgery. Multiple continuously monitored noninvasive hemodynamic values in the present study also provide an integrated approach to the cardiac, pulmonary, and tissue perfusion functions [111, 113, 119, 121–129].
Methods
Clinical Series
P&S monitoring and hemodynamic monitoring were performed on 208 consecutive severe septic patients (47 females, 22.6 %). A Student T-test was performed given the low number of females in this cohort. The T-test finds that the females’ results are statistically similar to the males (p = 0.007). Measurements were started shortly after admission to the emergency department. Patient selection criteria was temperature >38 °C or <36 °C; tachycardia (HR >110 beats/min); white blood cell count >12,000 or <3,000; and evidence of a septic focus or positive blood cultures. These were frequently accompanied by an episode of hypotension (systolic BP <100 mmHg or MAP <70 mmHg). During their current hospitalization, 160 patients survived; the mortality was 23 %. Table 29.5 lists demographic and salient clinical features. Children <17 years of age were excluded. The university’s Institutional Review Board approved the study.
Table 29.5
Salient features of the clinical series on sepsis
Age, years, mean ± SD | 39.7 ± 17.3 |
Gender, M/F | 161 (77 %)/47 (23 %) |
Survivors/non-survivors (% mortality) | 160/48 (23 %) |
ISS score | |
Survivors | 25.4 ± 11.2 |
Non-survivors | 31.5 ± 12.3 |
Study Design and Management Policies
Patients admitted to level one trauma center were managed by a dedicated full-time, 24 h/day, 7 days/week attending faculty and resident staff. Septic patients were treated in accordance with established protocols. Continuous, noninvasive, P&S and hemodynamic monitoring began shortly after admission to the emergency department and continued as the patient went to the radiology department, to the operating room, and then into the ICU. In the present study, we evaluated >40,000 values in >6,000 data sets in 208 patients with severe sepsis and septic shock. Invasive monitoring with pulmonary artery catheters was also used when indicated by clinical conditions after ICU admission.
P&S Monitoring
See Fig. 3.8 and Chap. 5 of this compendium for a complete methodology describing the Autonomic Assessment. This is a retrospective study. The temporal patterns of autonomic data were compared with simultaneously measured hemodynamic data. We separately evaluated the patients who survived hospitalization and those who died during their current hospitalization.
Invasive Hemodynamic and Oxygen Transport Monitoring
A pulmonary artery (PA) thermodilution catheter (Swan-Ganz®) was placed in high-risk patients on clinical indication after admission to the ICU. Cardiac output was measured by the standard thermodilution method. Arterial and mixed venous blood gas samples were sampled at the time of thermodilution measurement, immediately analyzed, and used to calculate oxygen delivery (DO2) and oxygen consumption (VO2) by standard formulas [111, 113]. Flow-related variables were indexed to body surface area.
Noninvasive Cardiac Output Monitoring
A thoracic bioelectric impedance device (IQ System, Noninvasive Medical Technologies, LLC, Las Vegas, NV, or the PhysioFlow, VasoCOM, Bristol, PA) is applied as soon as possible following ER admission. Four pairs of disposable prewired hydrogen electrodes were appropriately positioned on the skin, and the EKG leads were placed across the precardium and left shoulder [111, 123, 124]. At 100 kHz, the outer pairs of electrodes passed 4 mA alternating current through the patient’s thorax, and the inner pairs of electrodes measured the voltage difference. Baseline impedance (Zo) was calculated from the voltage changes sensed by the inner pairs of electrodes. The first derivative of the impedance waveform (dZ/dt) was calculated from the time-impedance curve. The digital signal processing used time-frequency distributions that increased the signal-to-noise ratio [111, 123, 124]. Measurements of cardiac index (CI), MAP, HR, pulse oximetry (SapO2), transcutaneous O2 (PtcO2) and CO2 (PtcCO2) tensions, and the fractional inspired oxygen concentration (FiO2) were continuously monitored, recorded by an interfaced personal computer, and directly filed in a database.
Blood Pressure and Heart Rate
MAP was measured with a digital cuff sphygmomanometer (Dinamap, Critikon, Tampa, FL). MAP was calculated by the device
and recorded at intervals simultaneously with the other values. HR was taken from EKG tracings [113].

Pulse Oximetry
A standard pulse oximeter (Nellcor, Pleasanton, CA) placed on a finger or toe in the routine fashion was used to measure arterial hemoglobin oxygen saturation (SapO2) continuously. Measurements were monitored continuously and recorded at intervals simultaneously with the other values. When there were major changes in pulse oximetry values, they were compared with arterial oxygen saturation obtained by routine blood gas analyses [113].
Transcutaneous O2 and CO2 Monitoring
The patients were continuously monitored with transcutaneous PtcO2 and PtcCO2 sensors (Novametrix Medical Systems, Wallingford, CT) in a standardized fashion. After cleaning the skin with alcohol, a gel electrolyte was applied to the sensor. The sensor was fixed by an adhesive ring the skin on the anterior chest wall or shoulder depending on area of injury and surgical procedure. A two-point gas calibration was done, and 20 min was allowed for the sensors to equilibrate. Every 4 h, the PtcO2 and PtcCO2 sensors were placed on a nearby skin location to avoid electrode-induced first-degree skin burns, recalibrated, and allowed to equilibrate [125–129]. PtcO2 was measured continuously, recorded at standard intervals, and filed directly into a database. PtcO2 values were measured in torr, indexed to FIO2, and expressed as the ratio PtcO2/FiO2. Transcutaneous carbon dioxide (PtcCO2) tension of the skin surface was monitored with the standard Stow–Severinghaus electrode [125, 126].
Statistics
The mean and SEM of each variable at comparable time periods after ER admission are calculated using the GraphPad Prism statistical program. For continuous data variables collected to be compared at comparable temporal conditions, we used the two-tailed Student t-test with the Bonferroni’s correction as well as Mann–Whitney U test. Differences are considered significant at probability values <0.05.
Results
Temporal P&S and Hemodynamic Patterns of Surviving and Non-surviving Septic Patients
Table 29.6 lists the mean ± SEM P&S and hemodynamic values for 140 surviving and 38 non-surviving patients with sepsis; the mortality was 21.3 %. Figure 29.5 illustrates the temporal patterns of these variables during the first 96 h after ER admission. Overall, non-survivor P&S activity (RFa and LFa, respectively) is higher than for the survivors (Table 29.6). However, only the parasympathetic differences between the non-survivors and survivors are significant (p = 0.012; for the sympathetics p = 0.270). The temporal P&S patterns, as presented in Fig. 29.5 (top panel presents the sympathetic (LFa) patterns and the second panel presents the parasympathetic (RFa) patterns), indicate two phases to the ER period. In the early period (the first 24 h), the non-survivor’s autonomic levels are significantly higher than the survivors’. In the later period (about 72 h after admission during the preterminal and terminal periods for the non-survivors), the reverse is the case, and the survivors’ autonomic levels are significantly higher than the non-survivors’. The non-survivors have near zero autonomic values. SB is significantly higher in the survivors throughout the entire ER period (Table 29.6).

Table 29.6
P and S and hemodynamic values in survivors and non-survivors of sepsis
Survivors | N | Non-survivors | N | p value | |
---|---|---|---|---|---|
Mean ± SEM | Mean ± SEM | ||||
LFa | 3.32 ± 0.62 | 55 | 4.78 ± 1.38 | 18 | 0.27a |
RFa | 4.83 ± 1.38 | 55 | 13.34 ± 4.64 | 18 | 0.012 |
L/R | 8.68 ± 2.11 | 55 | 1.23 ± 0.22 | 18 | 0.048 |
CI | 4.03 ± 0.02 | 140 | 3.75 ± 0.02 | 38 | 0.001 |
HR | 113 ± 1 | 140 | 112 ± 1 | 38 | 0.61a |
MAP | 85 ± 1 | 140 | 83 ± 1 | 38 | 0.29a |
SapO2 | 98.3 ± 1 | 140 | 97.7 ± 1 | 38 | 0.001 |
PtcO2/FiO2 | 175 ± 2 | 140 | 129 ± 3 | 38 | 0.001 |
DO2 | 623 ± 11 | 52 | 558 ± 17 | 22 | 0.006 |

Fig. 29.5
Temporal P and S and hemodynamic patterns in a series of 178 severely septic patients. The patterns of 140 survivors (solid line) and 38 non-survivors (dashed line) are shown. Note the LFa and RFa were higher in the non-survivors, while the CI, MAP, SapO2, and PtcO2/FiO2 ratio were higher in the survivors during the period of observation
Overall, the CI for survivors is higher than for non-survivors (Table 29.6: survivors=4.03 l/min/m2, non-survivors=3.75 L/min/m2; p = 0.001). In the early period, the survivors demonstrate a mean CI significantly higher than the non-survivors (Fig. 29.5, “CI”). After 72 h, the non-survivors’ CI rises and becomes significantly greater than the survivors’, but then the CI in non-survivors falls below the survivors’ returning to the normal range, and the survivors’ CI becomes high during this time. From the hemodynamic data, overall, HR is elevated throughout the ER period in both groups but greater in the non-survivors (Table 29.6 and Fig. 29.5). HR differences between the two groups are not significant (p = 0.610). Throughout the ER period, the mean arterial pressure (MAP), pulse oximetry (SapO2), and transcutaneous oxygen (PtcO2/FiO2) ratio (Table 29.6 and Fig. 29.5) are normal for the survivors and lower in the non-survivors. MAP differences between the two groups are not significant (p = 0.290). The SapO2 values are within normal limits for both groups throughout. HR and MAP differences between the two groups are not significant (p = 0.61 and, respectively). The differences for SapO2 and PtcO2/FiO2 ratio are significant (p = 0.001 for both). The PtcO2 is higher for the non-survivors throughout until the very end (Fig. 29.5). Overall, the DO2 is significantly higher in the survivors (Table 29.6, p = 0.006). Unlike the early to late ER period reversals seen in the autonomic and CI data, the HR, MAP, SapO2, PtcO2, and PtcO2/FiO2 differences are consistent throughout the ER period, except for PtcO2 at the very end.
Hemodynamic Changes During Periods of Sudden Changes in P&S Function
See section “Trauma” above for these results.
P&S and Hemodynamic Patterns of Postoperative Patients with Sepsis
Table 29.7 lists the values of 35 patients with postoperative sepsis. The preoperative, operative, and postoperative hemodynamic patterns are presented in Fig. 29.6. Focusing on the postoperative period, the data in Table 29.7 indicates that the survivors have higher average hemodynamic level (CI = 3.9, MAP = 87.0, and PtcO2/FiO2 = 179) values than did the non-survivors (CI = 3.5, MAP = 73.0, and PtcO2/FiO2 = 112; with respective significance values of 0.05, 0.001, and 0.001), and the non-survivors have higher P&S values (4.79 and 3.42, respectively) than the survivors (1.33 and 1.08, respectively; each with significance values of 0.001). Figure 29.6 presents the temporal hemodynamic patterns of these patients from preop through postop. Figure 29.6 shows that the non-survivors’ MAP and tissue saturation values (e.g., SapO2 and PtcO2/FiO2) throughout the entire postop period are lower than the survivors’. The temporal patterns for CI and HR are mixed between the survivors and non-survivors.

Table 29.7
P and S and hemodynamic values in survivors and non-survivors with sepsis as a complication of trauma
Survivors (N = 24) | Non-survivors (N = 11) | P value | |
---|---|---|---|
Mean ± SEM | Mean ± SEM | ||
LFa, bpm2 | 1.08 ± 0.12 | 3.42 ± 0.88 | 0.001 |
RFa, bpm2 | 1.33 ± 0.22 | 4.79 ± 0.98 | 0.001 |
L/R | 10.4 ± 2.52 | 1.2 ± 0.20 | 0.02 |
CI | 3.9 ± 0.03 | 3.5 ± 0.06 | 0.05 |
HR | 106 ± 1 | 104 ± 1 | ns |
MAP | 87 ± 1 | 73 ± 1 | 0.001 |
SapO2 | 98 ± 1 | 96 ± 1 | ns |
PtcO2/FiO2 | 179 ± 3 | 112 ± 5 | 0.001 |

Fig. 29.6
Hemodynamic patterns of patients who became septic postoperatively. Note the higher CI, HR, MAP, and PtcO2/FiO2 in the survivors with the onset of sepsis
Patterns of Sepsis as the Primary Etiologic Event and as a Secondary Complication
Figure 29.7 describes the temporal hemodynamic patterns of patients who on ER admission have sepsis from community-borne infections with no evidence of trauma. The survivors’ values are all higher than the non-survivors’. Table 29.8 presents the data of trauma patients who are documented immediately before and after onset of an acute septic complication. The survivors start with higher CI, HR, MAP, SapO2, and PtcO2/FiO2 levels than the non-survivors. The survivors’ levels after onset of sepsis are lower for CI, MAP, SapO2, and PtcO2/FiO2 and higher for HR than in the non-survivors. However, only the increased MAP in the survivors and the increased CI, MAP, and HR values in non-survivors are significant (p < 0.005; see Table 29.8).


Fig. 29.7
Temporal hemodynamic patterns of 16 patients who entered the hospital with community-borne infections showing increased CI, and PtcO2/FiO2 for the survivors, and an initially increased HR in the non-survivors
Table 29.8
Hemodynamic data of patients immediately before and after a septic complication of trauma
Survivors (N = 5) | Non-survivors (N = 4) | |||||
---|---|---|---|---|---|---|
Mean ± SEM | Mean ± SEM | |||||
Before | After | p-value | Before | After | p-value | |
CI | 4.24 ± 0.15 | 4.33 ± 0.13 | ns | 3.54 ± 0.04 | 4.42 ± 0.13 | 0.001 |
HR | 104 ± 3 | 101 ± 2 | ns | 92 ± 1 | 106 ± 3 | 0.004 |
MAP | 103 ± 2 | 87 ± 2 | 0.001 | 82 ± 2 | 68 ± 2 | 0.003 |
SapO2 | 98 ± 1 | 98 ± 0 | ns | 97 ± 1 | 94 ± 1 | ns |
PtcCO2 | 38 ± 1 | 43 ± 2 | ns | 60 ± 2 | 63 ± 2 | ns |
PtcO2/FiO2 | 163 ± 14 | 144 ± 5 | ns | 82 ± 8 | 60 ± 6 | ns |
Terminal Hemodynamic Patterns of Late Sepsis
Four septic patients are studied in their terminal states. Their hemodynamic patterns are characterized by rapidly decreasing CI, MAP, HR, SapO2, and PtcO2/FiO2 values (data not shown).
Discussion
It is known that sympathetic activity directly influences BP and HR. BP is influenced through the baroreceptor reflex and peripheral resistance, both of which are (in part) controlled by sympathetic activity. HR is controlled through pacing at the SA node. The sympathetics are known as the reactive branch of the ANS. For example, the SNS enables BP and HR changes that do not require basal metabolic changes. The parasympathetics are known to set the metabolic baseline, mediate healing and proper tissue perfusion (SapO2, PtcO2/FiO2, and DO2), and mediate rest and recovery. This dichotomy in function may describe the differences in outcomes attributed to normalizing HR and BP with and without normalizing the cumulative hemodynamic deficit [4, 115, 119, 121, 122, 126, 127, 129–134]. Of interest is how these relationships specifically present in our patient population. Figure 29.2 and Tables 29.7 and 29.9 seem to indicate that a direct interrelationship between P&S and hemodynamic activity exists.
Table 29.9
P and S and hemodynamic values at periods of reduced autonomic function
At maximum | Post-maximum baseline | Change | P value | |
---|---|---|---|---|
(N = 208) | (N = 208) | |||
Mean ± SEM | Mean ± SEM | Mean ± SEM | ||
LFa, bpm2 | 22.3 ± 8 | 1.8 ± 0.6 | −20.5 ± 7.6 | 0.01 |
RFa, bpm2 | 36.7 ± 16 | 3.8 ± 1.7 | −32.9 ± 15.4 | 0.04 |
L/R | 7.5 ± 5.4 | 6.7 ± 5.0 | −0.7 ± 0.9 | ns |
CI | 4.36 ± 0.27 | 3.52 ± 0.26 | −0.83 ± 0.16 | 0.03 |
HR | 114 ± 4.8 | 105 ± 5 | −9.0 ± 3 | 0.05 |
MAP | 92 ± 4 | 80 ± 4 | −12 ± 3 | 0.03 |
PtcO2/FiO2 | 163 ± 23 | 202 ± 21 | +39 ± 12 | 0.05 |
General Observations
Overall the survivor/non-survivor differences in the sympathetic measure (LFa, Table 29.6) are not significant (p = 0.27), but the differences in the parasympathetic measure (RFa, Table 29.6) are significant (p = 0.012). Of the two autonomic measures, this may suggest that the parasympathetics are more indicative of outcome. This seems to be supported by the hemodynamic data. Sympathetically mediated indices such as mean HR and MAP were not significantly different between survivors and non-survivors (p = 0.610 and p = 0.290, respectively). However, the parasympathetically mediated tissue perfusion indices were significantly different (p ≤ 0.006). Overall, the survivors of sepsis had greater average CI, HR, MAP, SapO2, PtcO2/FiO2, and DO2 than did the non-survivors. Previous studies document comparable thermodilution and bioimpedance cardiac index values under similar conditions in the ER, OR, and ICU [111, 113].
Early Versus Late ER Period
Upon closer inspection (Fig. 29.5), there seems to be temporal pattern differences between the survivors and non-survivors. There seems to be a distinction between the survivor/non-survivor P&S and hemodynamic levels in the early ER period (during the first 24 h after ER admission) and the later ER period (up to 96 h after ER admission). In the early ER period, non-survivors’ P&S values were higher than those of the survivors’ values (p < 0.001), indicating pronounced P&S activity at this time in the non-survivors. In the early ER period, CI and tissue perfusion differences between the two groups were significant (p < 0.001), and HR and MAP were not (p > 0.250). Since the parasympathetic and tissue perfusion indices were significantly different, perhaps together elevated parasympathetics with depressed tissue perfusion may be physiologic markers of the preterminal phase, possibly providing more time to attempt to recover the patient. In the later ER period, the non-survivors’ P&S activity indices dropped to near zero by 72 h after ER admission. Near zero P&S values suggest the loss of autonomic involvement in cardiac and pulmonary function. Very low parasympathetic measures are associated with cardiac autonomic neuropathy (CAN), which is associated with high mortality risk and high risk of sudden cardiac death [65, 118–120, 135]. The relationship between parasympathetic activity and CAN is independent of sympathetic activity, except that relative sympathetic activity (high SB) positively stratifies the risks [115]. This study shows that normalizing parasympathetic activity (normalizing SB) reduces or minimizes mortality risk.
Considering the early from the later ER periods, the present study indicates five results:
1.
The survivors of sepsis have greater CI, MAP, SaO2, PtcO2/FiO2, and DO2 than did the non-survivors.
2.
In the early period, non-surviving septic patients’ P&S values are higher, and the parasympathetics are significantly elevated compared with those of the survivors’. This may be because the non-survivors need more autonomic activity to compensate for their impaired hemodynamic status.
3.
Hemodynamic patterns appear to be regulated by sudden abrupt surges of both P&S activities.
4.
The cessation of the P&S surges is immediately associated with the opposite hemodynamic effect.
5.
Parasympathetic values are more elevated than the sympathetic values in sepsis.
These findings suggest that parasympathetic activity in septic patients is at least as important a biomarker for survival as sympathetic activity. These data support the finding that parasympathetic activity and tissue perfusion are significantly associated with survival. Sympathetic activity and mean HR and MAP are not. The parasympathetic significance reflects hemodynamic deficit. As long as the hemodynamic deficit persists, parasympathetic activity remains high, indicating that non-survivors are not unable to overcome [97, 101].
Role of the Parasympathetic Nervous System
P&S activities in surviving and non-surviving septic patients were similar to those of trauma patients without sepsis [4]. Increased parasympathetic tone was not unexpected in septic patients, since parasympathetic increases are known to be involved in the recovery process of cardiac patients [98–100]. Elevated SB results from increased sympathetic activity and is similar to the stress response to trauma due to uneven metarteriolar vasoconstriction and appears to be related to surges of sympathetic and parasympathetic tone [4]. Normalization of parasympathetic activity after sepsis, similar to trauma, leads to localized redistribution of flow from metarteriolar vasoconstriction [136–138]. The uneven metarteriolar vasoconstriction results in uneven tissue perfusion with local areas of reduced tissue perfusion and oxygenation. In shock, progressively increasing areas of flow maldistribution contribute to increasing tissue hypoxia that leads to global tissue hypoxia, a physiologic hallmark of shock [139]. There are also differences in flow (CI) and tissue perfusion (PtcO2/FiO2) between septic survivors and non-survivors after high-risk surgical operations.
High-risk surgery, trauma, and sepsis have been shown to stimulate hemodynamic function as indicated by increased CI, MAP, HR, DO2, and VO2 [113, 118–120, 137, 138, 140–148]. When trauma was complicated by sepsis, body metabolism increased, apparently associated with increased parasympathetic activity. The increases in CI, MAP, PtcO2/FiO2, DO2, and VO2 are evident in the survivors, suggesting that they are the result of compensatory responses with survival value.
The Terminal State
The terminal state in septic patients is marked by progressive fall in CI, MAP, DO2, VO2, and PtcO2/FiO2 values with increased HR, followed by bradycardia and increased PtcCO2 values. The PtcO2/FiO2 values of zero or near zero with high PtcCO2 values in extremely ill and dying patients are consistent with global tissue hypoxia.
Gender Differences
P and S changes associated with sepsis show gender differences. In the early ER period, female non-survivors had greater parasympathetic activity, while male survivors had greater sympathetic activity. Non-survivors’ increases of both genders occurred early, i.e., in the first day of admission. In survivors, these P&S patterns were associated with increased CI and HR, normal MAP, SapO2, and PtcO2/FiO2. Non-survivors had normal CI, hypotension, tachycardia, low PtcO2/FiO2 and SapO2, and reduced oxygen delivery. Increased autonomic activity was greater in females and greater in those who died, especially females. Overall, the salient difference between survivors and non-survivors was parasympathetic activity [149].
Sympathovagal Balance
In all 208 patients, SB was markedly abnormal, and they demonstrated P&S imbalance. In the patients with poor outcomes, their P&S imbalance persisted. These patients also had low MAP, CI, and PtcO2/FiO2. Patients whose SB was normalized early in their ED stay all survived. Patients whose SB was improved latter in their ED stay showed mixed results, but generally had improved outcomes. SB was not necessarily well correlated with general hemodynamic measures, especially HR, BP, and CI [116].
Conclusion
The data presented herein seem to support the theory that there exists a dichotomy between sympathetic activity and sympathetically mediated hemodynamic activity (HR and MAP) on the one hand and parasympathetic activity and parasympathetically mediated hemodynamic activity (SapO2, PtcO2/FiO2, and DO2) on the other. This study finds that:
1.
The survivor/non-survivor sympathetic data are not significantly different.
2.
The survivor/non-survivor sympathetically medicated hemodynamic data are also not significantly different.
3.
The survivor/non-survivor parasympathetic data are indeed significantly different.
4.
The survivor/non-survivor parasympathetically medicated hemodynamic data are also significantly different.
These significances are present overall and during the early and later ER periods and seem to be correlated with outcome. These differences may be indicative of a causal relationship between the two systems.
P&S monitoring data are consistent with the concept that increased P&S values occur with sepsis as well as trauma and surgery. The data also suggest that increased autonomic activity is an important regulatory mechanism for the developing hemodynamic patterns. However, intense sympathetic activity may lead to uneven metarteriolar vasoconstriction that alters the distribution of microcirculatory flow and may limit local tissue oxygenation. Tissue perfusion/oxygenation depends on both the overall blood flow (CI), as well as the even distribution of microcirculatory blood flow needed to oxygenate local tissues. PtcO2/FiO2, which is a direct measure of local tissue oxygenation, may be a useful marker of the adequacy of tissue perfusion/oxygenation in septic and posttraumatic states [130, 138].
Hypovolemia
The following are excerpts from a Navy Report documenting a critical care study funded by the Office of Naval Research in the area of Combat Casualty Care [150].
P&S monitoring may be used as a continuous or periodic measure of health. The Navy is interested in the technology for, among other issues, Combat Casualty Care applications where hypovolemia is a significant issue. The technology’s digital, real-time capabilities offer solutions for (1) continuous, remote monitoring of deployed personnel; (2) automated, continuous, remote triage from forward areas to field hospitals, to hospital ships (e.g., through all levels of care: E1 through E3), including the transit periods; and (3) intraoperative care including preoperative care, surgical and anesthetic interventions, postoperative care, and recuperation.
Background
This study considers a variety of anesthesia and surgical intervention effects on the patient as measured by P&S monitoring. Data were collected during the immediate intraoperative period. The anesthesia and surgical intervention effects include (1) preoperative, post-induction baseline; (2) incision; (3) unknown events; (4) bleeding; (5) additional sedation; and (6) intra-operation stable. These effects are listed in chronological order from immediately following entry into the operating room to immediately prior to the patient’s departure from the operating room.
Methods
Patient Selection
Patients were selected for study in the operating room based on their potential for significant hemorrhage, the ability to apply the monitor leads on either side of the chest, and the presence of the clinical investigator. Major abdominal, spinal, and acetabular surgery provided the most consistent volume of hemorrhage among study patients. Although the average blood loss from a femur fracture is 1,000 ml, most of this occurs at the time of injury and resolves spontaneously prior to the time of surgical repair. A couple of femur fractures were included early in the study, but cases with more intraoperative blood loss were sought for the remainder of the study.
The ability to apply the P&S monitor to patients with ongoing hemorrhage from acute traumatic injuries was hampered. Monitor leads could not be placed laterally on the chest, because of the normal, wide thoracic–abdominal surgical preparation required for undiagnosed injuries. Although EKG leads will function at more distant sites, the impedance measurement of respiratory rate will not. Also, the clinical investigator was frequently unable to take the time to get the P&S monitor and hook it up in the midst of emergency surgery. This is a common flaw in emergency research protocols. Future studies in the acute care setting should include personnel specifically dedicated to completion of the study. Another solution would be to integrate the P&S monitoring technology into the surgical suite’s central station monitor.
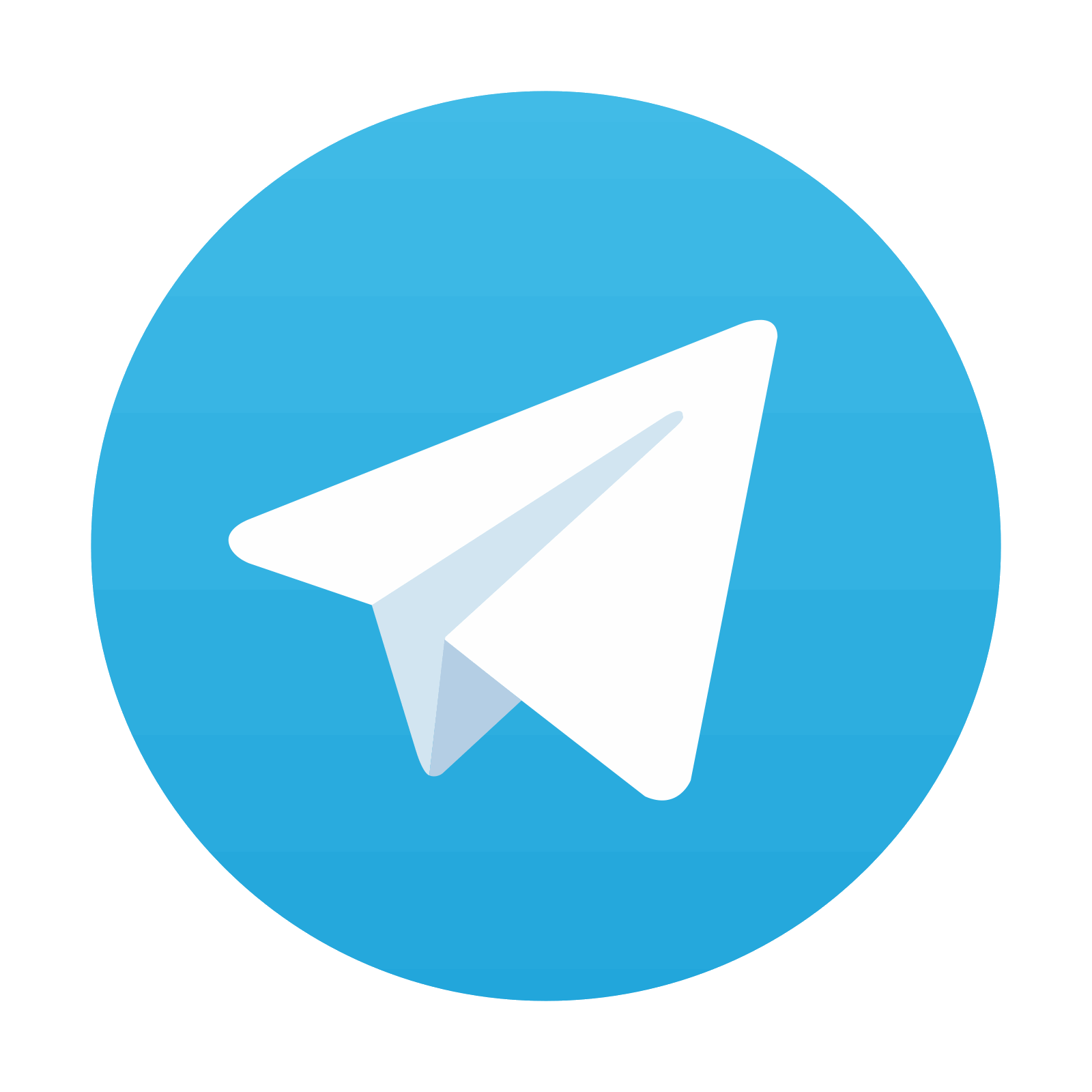
Stay updated, free articles. Join our Telegram channel
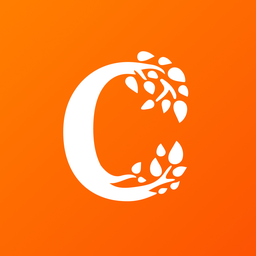
Full access? Get Clinical Tree
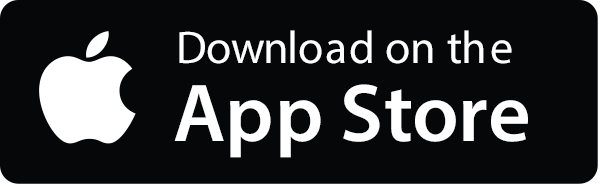
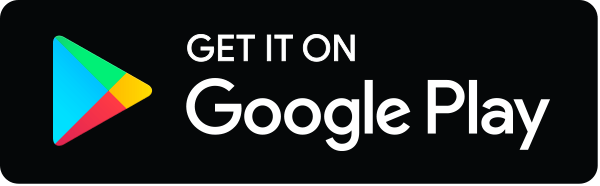
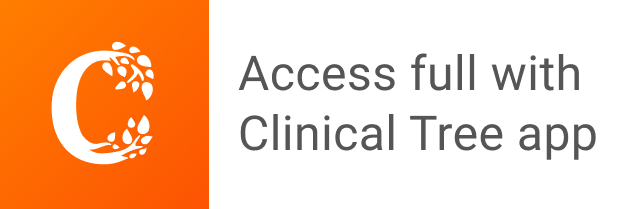