Coronary Magnetic Resonance Angiography: Technical Approaches
Oliver M. Weber
Coronary artery disease is one of the major causes of morbidity and mortality in the Western world, where it is responsible for about one-sixth of all deaths (1). The current gold standard for the diagnosis of coronary disease is selective x-ray coronary angiography. Even though the number of procedures performed has slightly declined over recent years, more than 1,000,000 of these diagnostic procedures are performed each year in the United States (1) and Europe. X-ray coronary angiography is used to define coronary anatomy and guide patient therapy. However, x-ray coronary angiography is expensive and invasive, exposes both patient and operator to potentially harmful ionizing radiation, and carries a small risk for serious complications. Furthermore, a significant minority of patients undergoing x-ray angiography are found not to have any significant disease (2), but remain exposed to the costs and risks of this invasive procedure. Thus, a more cost-effective, noninvasive approach for defining luminographic disease is urgently needed.
Multidetector computed tomography (MDCT) has recently gained great popularity and experienced great success in clinical use for coronary angiography (3). Three-dimensional (3D) data sets can be obtained during intravenous injection of contrast media in a single breathhold. The diagnostic accuracy for the detection of significant stenoses is good, showing a high negative predictive value and a somewhat lower positive predictive value (3). Recent technical developments such as dual-source CT and higher rotation speed of the gantry, as well as iterative reconstruction algorithms, eliminated a substantial part of the problems previously encountered in patients with higher heart rates or severe calcifications and may be expected to further improve the diagnostic accuracy (4). MDCT has therefore been found to be an appropriate method in the diagnosis of coronary artery disease in a number of clinical indications (5). However, despite recent efforts to reduce doses, MDCT exposes the patients to significant levels of ionizing radiation, which bears potential long-term risk (6). In some patients, beam-hardening effects, caused by metallic implants or severe calcifications, continue to cause severe image artifacts (7).
Coronary magnetic resonance angiography (MRA) combines several advantages and great potential. It is noninvasive and can survey the heart in arbitrary image planes, and it does not involve the use of possibly harmful ionizing radiation or iodinated contrast media. In addition to providing a high degree of spatial resolution, magnetic resonance (MR) is not associated with any known short- or long-term side effects. The utility of MRA for visualizing the coronary anatomy has been investigated since the late 1980s (8,9). Although no coronary stenoses were identified in these early studies, demonstrations of the potential of magnetic resonance imaging (MRI) to assess the anatomy of the coronary vessels triggered intense and ongoing interest in the field.
Successful coronary MRA data acquisition is technically demanding because of the small caliber and tortuosity of the coronary arteries and the presence of signal from surrounding epicardial fat and myocardium. In addition, cardiac and respiratory motion affects the position of the coronary arteries by a multiple of their diameter. Efficient strategies to suppress motion must therefore be applied. Furthermore,
enhancement of the contrast between the coronary vessel lumen and the surrounding tissue (myocardium, epicardial fat) is mandatory for a successful visualization of the coronary anatomy. Despite considerable technical progress and promising early clinical results, coronary MRA has not yet found widespread clinical acceptance, and is currently considered appropriate only in the evaluation of suspected coronary anomalies, but not in the evaluation of chest pain syndrome (5).
enhancement of the contrast between the coronary vessel lumen and the surrounding tissue (myocardium, epicardial fat) is mandatory for a successful visualization of the coronary anatomy. Despite considerable technical progress and promising early clinical results, coronary MRA has not yet found widespread clinical acceptance, and is currently considered appropriate only in the evaluation of suspected coronary anomalies, but not in the evaluation of chest pain syndrome (5).
The general approaches described in this chapter are available on current state-of-the-art cardiac MR units from all vendor platforms, but some nuances may be vendorspecific (e.g., navigator implementation). Established and advanced coronary MRA methods are reviewed. Specific strategies for motion suppression and contrast enhancement are discussed, and representative image material is displayed.
TECHNICAL CONSIDERATIONS
The main part of research studies and method development in coronary MRA has been conducted on 1.5-Tesla (T) whole-body systems. More recently, many of these methods were implemented and investigated on 3-T systems as well with the goal to exploit the higher signal-to-noise ratio (SNR) available at higher field strength (10,11). However, highquality coronary MRA data have also been obtained at lower field strength on 0.5-T systems (12,13 and 14).
SUPPRESSION OF MOTION ARTIFACTS
The heart is subject to intrinsic and extrinsic motion. Intrinsic myocardial motion is the rhythmic contraction and relaxation during the R-R interval. Extrinsic myocardial motion is the bulk motion of the heart induced by respiration. Both extrinsic and intrinsic myocardial motion may greatly exceed the coronary artery diameter and so cause blurring and ghosting of the coronary vessels on images. Therefore, strategies that minimize the adverse effects of both motion components are needed. Suppression of intrinsic motion is routinely achieved by synchronization with the electrocardiogram. Methods for the suppression of extrinsic motion include patient breath-holds, signal averaging to reduce the effects of respiration, and detection of respiratory status by external sensors such as respiratory belts or by means of MR methods.
Suppression of Intrinsic Myocardial Motion
For submillimeter coronary MRA, the image data cannot be collected during a single R-R interval. Therefore, the coronary MRA data acquisition must be synchronized with the cardiac cycle, and k-space segmentation must be applied (Fig. 20.1). For segmented k-space techniques, an accurate electrocardiographic (ECG) synchronization is mandatory. Less robust peripheral pulse detection methods yield inferior results. Even though ECG triggering is superior to peripheral pulse detection, reliable R-wave detection in the presence of a strong static magnetic field (causing the “hydrodynamic effect”) and switching magnetic field gradients is technically challenging. A four-lead vector ECG approach has been shown to provide more robust ECG triggering than three-lead recording (15), especially at higher magnetic field strengths, and signal filtering can further improve the triggering quality.
During the cardiac cycle, the coronary arteries can move more than 1 cm, a multiple of their own diameter (Fig. 20.2).
The displacement of the right coronary artery (RCA) is larger than that of the left anterior descending (LAD) or the left circumflex (LCX) artery. The coronary arteries are relatively quiescent for short periods after completion of ventricular systole and, for a longer period, at mid-diastole (16,17). To minimize motion artifacts in the images, the coronary MRA data should be acquired during a period of minimal myocardial motion. Mid-diastole offers a longer acquisition opportunity and represents a period of rapid coronary blood flow resulting in inflow of unsaturated spins, a major mechanism of contrast in many bright blood (gradient-echo) sequences. Both duration and time point after R-wave of the quiescent phases can be roughly estimated based on simple models and by taking into account the patient’s heart rate. However, both values are strongly subject-specific (17), and for optimal results, individual trigger delays and optimal acquisition windows may thus have to be defined by visual inspection of cine images or more advanced automated approaches (18,19 and 20). Selection of the acquisition window duration is a tradeoff between the competing interests to freeze coronary motion (requiring a short acquisition window) and to achieve an acceptable overall acquisition duration (requiring a long acquisition window). The acquisition window in each R-R interval should therefore be chosen as long as the quiescent phase. Depending on the sampling strategy in k-space, it can also be chosen slightly longer without negative effect on the sharpness of the resulting images. Applying intra-RR motion correction based on partial data sets and motion correction during postprocessing, the acquisition window could be extended even further, cutting the total acquisition time in half (21).
The displacement of the right coronary artery (RCA) is larger than that of the left anterior descending (LAD) or the left circumflex (LCX) artery. The coronary arteries are relatively quiescent for short periods after completion of ventricular systole and, for a longer period, at mid-diastole (16,17). To minimize motion artifacts in the images, the coronary MRA data should be acquired during a period of minimal myocardial motion. Mid-diastole offers a longer acquisition opportunity and represents a period of rapid coronary blood flow resulting in inflow of unsaturated spins, a major mechanism of contrast in many bright blood (gradient-echo) sequences. Both duration and time point after R-wave of the quiescent phases can be roughly estimated based on simple models and by taking into account the patient’s heart rate. However, both values are strongly subject-specific (17), and for optimal results, individual trigger delays and optimal acquisition windows may thus have to be defined by visual inspection of cine images or more advanced automated approaches (18,19 and 20). Selection of the acquisition window duration is a tradeoff between the competing interests to freeze coronary motion (requiring a short acquisition window) and to achieve an acceptable overall acquisition duration (requiring a long acquisition window). The acquisition window in each R-R interval should therefore be chosen as long as the quiescent phase. Depending on the sampling strategy in k-space, it can also be chosen slightly longer without negative effect on the sharpness of the resulting images. Applying intra-RR motion correction based on partial data sets and motion correction during postprocessing, the acquisition window could be extended even further, cutting the total acquisition time in half (21).
Suppression of Extrinsic Myocardial Motion
BREATH-HOLD TECHNIQUES. Among the major difficulties encountered in coronary MRA is the bulk cardiac motion associated with respiration (Fig. 20.3). Early compensation of respiratory motion consisted of breath-holding (22). Two-dimensional (2D) breath-hold coronary MRA relied on the acquisition of contiguous parallel images, with the goal of surveying the proximal segments of the coronary arteries during serial breath-holds (23). Subsequently, 3D breath-hold techniques for coronary MRA were implemented (24,25 and 26). In combination with parallel imaging, even whole-heart coverage can be achieved, however, with the compromise of reduced spatial resolution, an increased acquisition window, and a breath-hold duration that is beyond the capacity of most patients (27).
Breath-hold approaches offer the advantage of rapid imaging and are technically easy to implement in compliant patients. However, they also have several practical limitations. Major patient and operator involvement is required for serial
breath-holds. Patients with cardiac or pulmonary disease frequently have difficulty sustaining adequate breath-holds, particularly when the duration exceeds 5 to 10 seconds. For sufficient anatomic coverage, a considerable number of serial breath-holds is often needed. Alternative breath-holding techniques, including serial brief breath-holds (28) and coached breath-holding with visual or audible feedback (29,30 and 31), have been used to minimize respiratory motion artifacts and patient inconvenience, but these are practical only for highly motivated subjects. Even with cooperative patients, breath-holding may be problematic. With a sustained breath-hold, cranial diaphragmatic drift, which is often substantial (˜1 cm), may occur (22,32,33 and 34). During serial breath-holds, the diaphragmatic and cardiac positions frequently vary by up to 1 cm, so that image registration errors result unless they are corrected for (35). Misregistration causes apparent gaps between the segments of the visualized coronary arteries, which can be misinterpreted as signal voids from coronary stenoses. Finally, breath-holds of reasonable duration (15 seconds) severely limit the options for improving spatial resolution, SNR, and volume coverage.
breath-holds. Patients with cardiac or pulmonary disease frequently have difficulty sustaining adequate breath-holds, particularly when the duration exceeds 5 to 10 seconds. For sufficient anatomic coverage, a considerable number of serial breath-holds is often needed. Alternative breath-holding techniques, including serial brief breath-holds (28) and coached breath-holding with visual or audible feedback (29,30 and 31), have been used to minimize respiratory motion artifacts and patient inconvenience, but these are practical only for highly motivated subjects. Even with cooperative patients, breath-holding may be problematic. With a sustained breath-hold, cranial diaphragmatic drift, which is often substantial (˜1 cm), may occur (22,32,33 and 34). During serial breath-holds, the diaphragmatic and cardiac positions frequently vary by up to 1 cm, so that image registration errors result unless they are corrected for (35). Misregistration causes apparent gaps between the segments of the visualized coronary arteries, which can be misinterpreted as signal voids from coronary stenoses. Finally, breath-holds of reasonable duration (15 seconds) severely limit the options for improving spatial resolution, SNR, and volume coverage.
SIGNAL AVERAGING AND RESPIRATORY BELTS. Initial freebreathing coronary MRA approaches used signal averaging to minimize motion artifacts (36,37). This averaging approach is reasonable for relatively low spatial resolutions (>2-mm pixel size), but inadequate for reliable detection of stenoses. As an alternative early free-breathing approach, thoracic respiratory belts were used to monitor chest wall expansion and thereby gate image acquisition to the end-expiratory position. Results were promising in comparison with breath-holding (34,38). Enhancements included respiratory feedback monitoring (31). However, respiratory belt gating is often not reliable due to poor correlation between abdominal motion and bulk motion of the heart. A time delay between chest wall expansion and diaphragmatic motion may occur, introducing additional motion artifacts to the images. Subsequently, more accurate and flexible MR navigators have replaced belt gating.
FREE-BREATHING NAVIGATOR APPROACHES. The use of free breathing with respiratory navigators, first proposed by Ehman and Felmlee (38), serves to overcome the time constraints and cooperation imposed by breath-hold approaches. Free-breathing navigator methods are particularly well suited for prolonged 3D coronary MRA approaches, which combine the benefits of thin, adjacent slices with the submillimeter spatial resolution afforded by an improved SNR. The use of navigator gating has received considerable attention, and implementations vary from relatively simple retrospective gating based on a single right-diaphragm navigator (39) to complex prospective affine motion correction based on multiple navigator signals (40). In principle, the MR navigator monitors the motion of an interface such as the lung-diaphragm interface (Fig. 20.4) or the lung-myocardium interface. Data are accepted only when the selected interface falls within a user-defined window (usually 3 to 7 mm) positioned around the end-expiratory level of the interface (Fig. 20.5). Although the need for patient cooperation and operator involvement is reduced with navigator/free-breathing methods, diaphragmatic drift and patient motion remain relevant issues (34,41). They may be taken into
account by manual or automated adjustment of the position of the acceptance window.
account by manual or automated adjustment of the position of the acceptance window.
Navigator Localization and Geometry. Navigators can be positioned at any interface that accurately reflects respiratory motion, including the dome of the right hemidiaphragm (30,37,42), the left hemidiaphragm, and the anterior chest wall, or directly through the anterior free wall of the left ventricle (34,43). Navigators have been implemented as two intersecting planes (44,45) and as 2D selective pencil beam excitations (46). Although the intersecting planes are easier to implement, they may compromise magnetization in the volume of interest due to saturation effects. In contrast, 2D selective pencil beam excitations can be implemented with the use of shallow radiofrequency (RF) excitation angles, so that they only minimally affect the magnetization in the region of interest. Studies suggest that cardiac motion related to respiration is predominantly in the superior-inferior axis (47,48) and that most single-navigator locations yield similar image quality (34,43). The right hemidiaphragm is therefore the preferred location for the navigator because of the relative ease of identifying the interface from a series of coronal, sagittal, and transverse scout images (Fig. 20.4).
Navigator Gating. The gating process can be retrospective or prospective. In retrospective gating, actual MRA data are recorded along with the respiratory position they are acquired in. After completion of data acquisition, only data segments acquired in a narrow acceptance window are used for the reconstruction of the MRA images. To ensure availability of all data segments at an acceptable respiratory position, k-space must be oversampled several times. In prospective gating, data segments are stored only if they are acquired in an acceptable respiratory position. Otherwise, the pulse sequence is played out (to maintain steady state), but data are not recorded. Exactly one copy of each data segment is thus acquired at an acceptable position, making this approach more efficient than retrospective gating. With navigator gating (without tracking), a 3-mm end-expiratory diaphragmatic window is typically used, and data are collected on average from one-third of R-R intervals (33% navigator efficiency) (34).
Navigator Gating and Tracking. From several MR studies, it was noted that the dominant impact of respiration on cardiac position is in the superior-inferior direction (47,48). The correction factor between diaphragm displacement and craniocaudal displacement of the RCA and left coronary artery (LCA) was early on reported to be approximately 0.6 and 0.7, respectively (47). Newer results suggest that the actual factors might be smaller and show considerable intersubject variability and that motion along the other two axes might not be negligible (48). Nevertheless, a great number of successful studies were performed with a displacement correction factor of 0.6 in craniocaudal direction only. Knowledge of this relationship offers the opportunity for prospective navigator gating with real-time tracking of the imaged volume position (42). This facilitates the use of wider gating windows and shortens scan time through increased navigator efficiency. The correction of the imaged volume position is obtained by a prospective run-time adaptation of the frequency of the slice-selective RF excitation and/or of the demodulator phase and frequency. With real-time tracking, a 5-mm diaphragmatic gating window is often used with a navigator efficiency close to 50% (49). Coronary MRA with real-time navigator tracking has been shown to minimize registration errors (in comparison with breath-holding), and image quality is maintained or improved in both 2D and 3D approaches (34,43).
A more elaborate tracking algorithm proposed by Manke et al. (40) accounts not only for craniocaudal translation but also for translation in the other two directions, as well as rotation, scaling, and shear transformation (summarized as affine transformation). Transformation parameters are first determined in a low-resolution dynamic scan during free breathing, with simultaneous recording of one to three navigator signals. During the high-resolution coronary scan, the imaged volume is then prospectively adapted to compensate motion. The affine motion model offers the potential to increase the navigator acceptance window and thus to increase the scan efficiency.
Navigator Tracking Alone. Limitations of breath-hold techniques include diaphragmatic drift and inconsistent end-expiratory positions between serial breath-holds. To overcome these limitations, breath-hold coronary MRA has been combined with navigator tracking (50,51). With these techniques, coronary MRA data can be acquired in serial breath-holds, and adverse effects of diaphragmatic drift during or between serial breath-holds can be minimized, while maintaining the high data acquisition efficiency of a breath-hold. Breath-holding with tracking during acquisition of central k-lines and subsequent free breathing with gating and tracking during acquisition of outer k-space data were also successfully combined to accelerate data acquisition or for use in combination with contrast media (52).
Advanced Navigator Techniques. Sophisticated navigator algorithms have been implemented to collect important k-space profiles more efficiently based on the navigator-detected interface position. Implementations of such k-space-reordered techniques include motion adaptive gating (53), the diminishing variant algorithm (54), phase ordering with automated window selection (55), and the zonal motion adaptive reordering technique (56).
Image-based Motion Compensation. As an alternative to assess diaphragm motion, a number of techniques have been presented that determine the position of the heart in each cardiac beat based on MR signal originating from the heart itself. These techniques include single-shot low-resolution images acquired in real time (57), tracking of epicardial fat (58), or determination of heart position from changes in the gradient-echo (59,60). All these techniques have in common that they do not rely on a model for the correlation between respiratory motion and heart motion. Instead, they assess directly the position of the heart, which allows for more accurate gating and tracking. These methods are thus more efficient in data collection and result in shorter acquisition duration.
Navigators, Prepulses, and Imaging Sequences. Most of the navigator concepts described can be freely combined with prepulses and 2D or 3D imaging sequences (Fig. 20.6). It has been found that navigator accuracy is improved by
preceding it with a fat saturation pulse because of reduced effect of the excitation sidebands. To provide optimal fat suppression during acquisition of the coronary MR data, a second fat suppression pulse immediately preceding data acquisition is beneficial. Since the navigator data are intended to reflect the position of the heart during the subsequent acquisition period, a short delay between the navigator and the data acquisition block and rapid navigator analysis is crucial (61). Some prepulses preceding the navigator excitation, such as nonselective inversion or dual-inversion pulses, compromise navigator detection of the interface position. Countermeasures such as local reinversion of the magnetization after the inversion or dual-inversion prepulses (Nav-Restore) were developed and successfully applied (62).
preceding it with a fat saturation pulse because of reduced effect of the excitation sidebands. To provide optimal fat suppression during acquisition of the coronary MR data, a second fat suppression pulse immediately preceding data acquisition is beneficial. Since the navigator data are intended to reflect the position of the heart during the subsequent acquisition period, a short delay between the navigator and the data acquisition block and rapid navigator analysis is crucial (61). Some prepulses preceding the navigator excitation, such as nonselective inversion or dual-inversion pulses, compromise navigator detection of the interface position. Countermeasures such as local reinversion of the magnetization after the inversion or dual-inversion prepulses (Nav-Restore) were developed and successfully applied (62).
CONTRAST ENHANCEMENT
The coronary arteries are surrounded by both epicardial fat and myocardium. For successful visualization of the coronary arteries, a high level of contrast between the coronary lumen and the surrounding tissue is desirable. The contrast between the coronary blood pool and the surrounding tissue can be manipulated by using the inflow effect (unsaturated protons entering the imaging field between successive RF pulses), by the application of MR prepulses (endogenous contrast enhancement), or by the administration of contrast agents (exogenous contrast enhancement) with or without preparatory pulses. Preparatory pulses such as fat saturation (63), magnetization transfer contrast (36), T2 preparation (64,65), local saturation bands, and inversion (66,67) and dual-inversion (68) prepulses were all shown to enhance contrast in coronary MRA.
Endogenous Contrast Enhancement
FAT SATURATION IN BRIGHT BLOOD CORONARY MAGNETIC RESONANCE ANGIOGRAPHY. In most subjects, the coronary arteries are surrounded by epicardial fat. Fat has a relatively short T1 (250 milliseconds at 1.5 T and 400 milliseconds at 3 T) and a resultant MR signal intensity similar to that of flowing blood. Fat saturation prepulses are used to suppress signal from surrounding fat selectively to allow visualization of the underlying coronary arteries. This is often accomplished with a frequency-selective prepulse that minimizes the fat signal and thereby allows visualization of the coronary vessels. Alternatively, a frequency-selective RF pulse, aiming at exciting exclusively the water resonance, may be used. However, for conventional segmented gradient-echo sequences, the duration of these pulses is too long for practical use. On the other hand, in the case of spiral imaging, typically, only a single RF pulse is applied in each heartbeat. In this case a spectral-spatial excitation RF pulse targeted at water can be used efficiently (69).
SIGNAL FROM THE MYOCARDIUM IN BRIGHT BLOOD CORONARY MAGNETIC RESONANCE ANGIOGRAPHY. The coronary arteries run in close proximity to the epimyocardium. The relatively similar T1 relaxation values of myocardium and coronary blood (1,000 and 1,200 to 1,400 milliseconds, respectively, at 1.5 T; 1,450 and 1,550 to 1,900 milliseconds at 3 T, respectively (70)) complicate the differentiation of the coronary arteries for 3D coronary MRA because blood exchange (inflow effect) is reduced between successive RF excitations as compared to 2D acquisitions. Different methods can be used to enhance the contrast between the coronary arteries and myocardium. The most frequently used ones are prepulses such as T2 preparation (64,65) and magnetization transfer contrast (36). Since the T2 relaxation times of coronary arterial blood (250 to 300 milliseconds) and myocardium
(40 to 50 milliseconds)) are substantially different, the application of a T2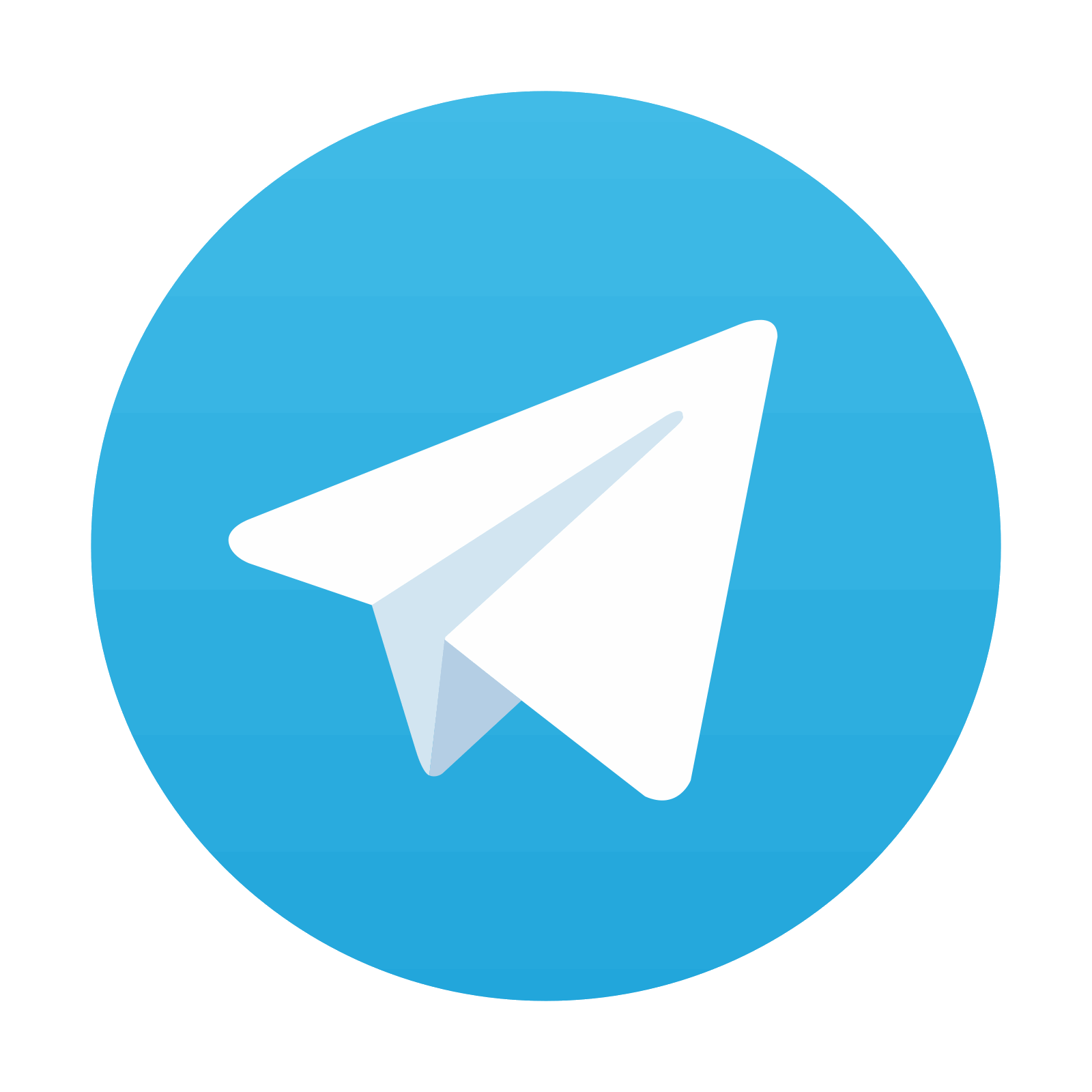
(40 to 50 milliseconds)) are substantially different, the application of a T2
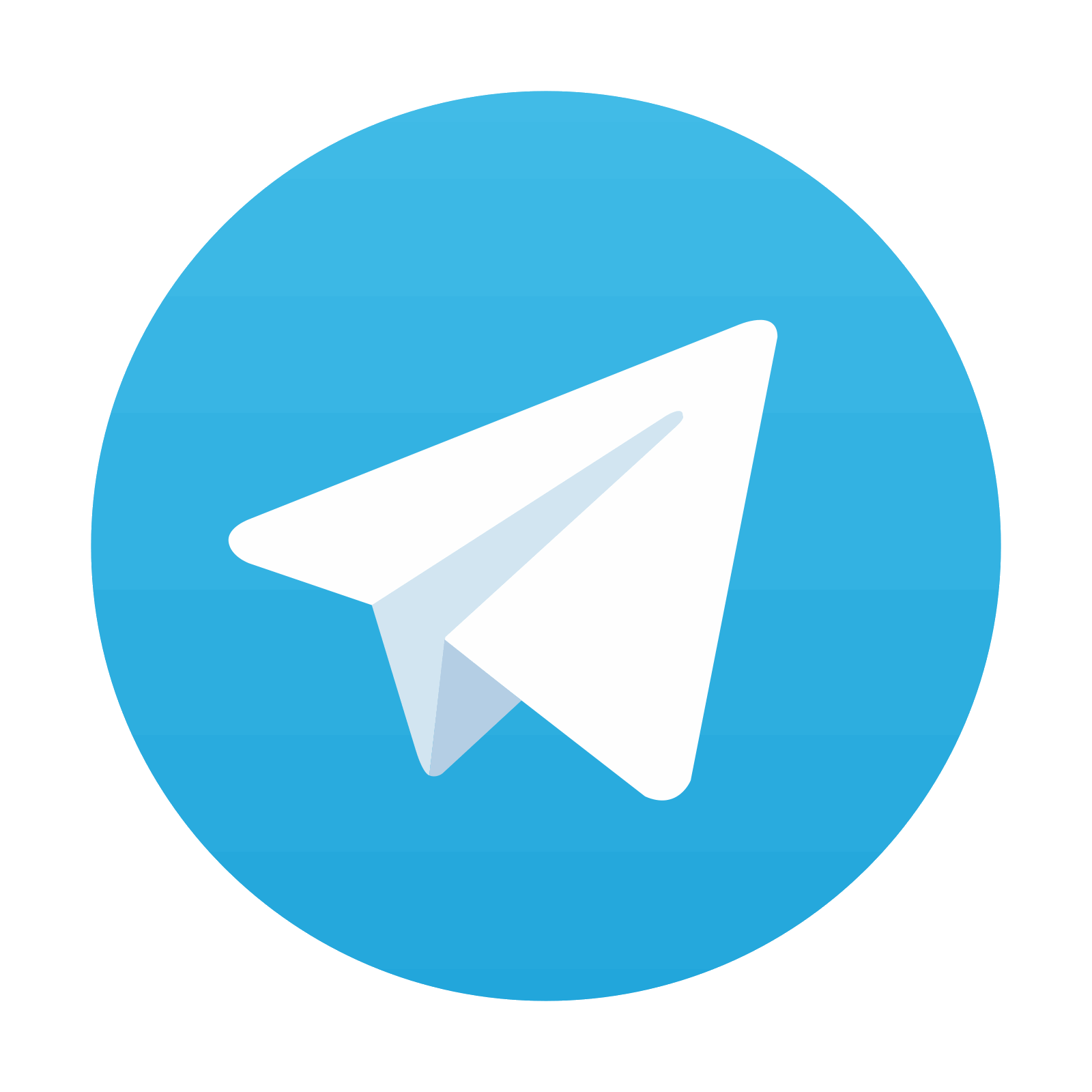
Stay updated, free articles. Join our Telegram channel
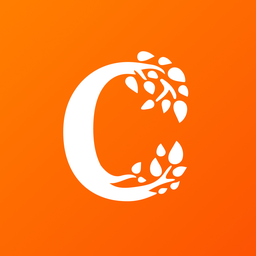
Full access? Get Clinical Tree
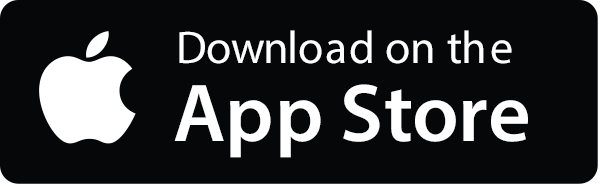
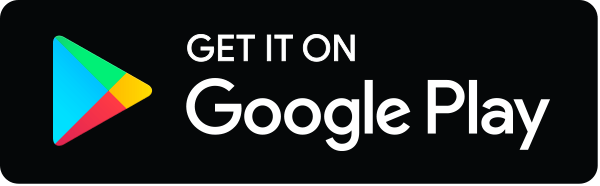
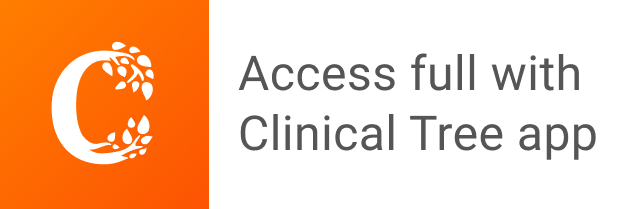