8
Coronary Artery Disease
Basic Principles of Stress Echocardiography
Dobutamine Stress Echocardiography
Limitations and Technical Aspects
END-STAGE ISCHEMIC CARDIAC DISEASE
Basic Principles
Coronary anatomy varies to some degree from patient to patient, but the overall pattern of coronary artery branching is relatively uniform (Fig. 8-1). The left main coronary artery arises from the superior aspect of the left coronary sinus of Valsalva and divides into (1) the left anterior descending (LAD) artery, which extends via the interventricular groove down the anterior wall to (and sometimes around) the LV apex and (2) the circumflex (Cx) artery, which continues laterally in the atrioventricular groove. The right coronary artery (RCA) arises from the superior aspect of the right coronary sinus of Valsalva and extends inferomedially following the atrioventricular groove. Approximately 80% of patients have a right-dominant coronary circulation; the right coronary artery gives rise to the posterior descending artery (PDA), which lies in the inferior interventricular groove. In about 20% of patients the coronary circulation is left dominant; the circumflex artery gives rise to the posterior descending artery.
Figure 8–1 Normal coronary anatomy.
A right anterior oblique angiogram shows the left anterior descending (LAD) and circumflex (Cx) coronary arteries (A). The right coronary artery (RCA) with the posterior descending artery (PDA) is seen in a right anterior oblique projection (B).
Segmental wall motion abnormalities seen by echocardiography correspond closely with the coronary artery blood supply to the myocardium (Fig. 8-2). The left anterior descending artery supplies the anterior portion of the interventricular septum via septal perforating branches and the anterior wall via diagonal branches. The posterior descending artery supplies the inferior aspect of the ventricular septum and the inferior free wall. The lateral wall is supplied by obtuse marginal branches of the circumflex artery. The posterior LV wall may be supplied by extension branches from the right coronary artery or by obtuse marginal branches of the circumflex arteries. There is marked individual variability in the blood supply to the LV apex. In some cases, the left anterior descending artery extends around the apex to supply the apical segment of the inferior wall. In other cases, the posterior descending artery extends around the apex to supply the apical segment of the anterior wall. More commonly, the blood supply to the apex arises from both the left anterior descending and the posterior descending coronary arteries.
Figure 8–2 Typical myocardial segments supplied by the right coronary artery
(RCA), left anterior descending artery (LAD), and circumflex (Cx) coronary arteries. The coronary anatomy is shown on the left with the corresponding wall segments in standard echocardiographic views on the right. The arterial distribution varies between patients. Some segments have variable coronary perfusion as indicated by the hatched regions. (From Lang RM, Bierig M, Devereux RB, et al: Recommendations for chamber quantification: A report from the American Society of Echocardiography’s Guidelines and Standards Committee and the Chamber Quantification Writing Group, developed in conjunction with the European Association of Echocardiography, a branch of the European Society of Cardiology. J Am Soc Echocardiogr 18:1440-1463, 2005.)
Standardized nomenclature for tomographic imaging of the heart allows consistency of terms, correlations between different imaging techniques, and a known relationship of each segment to coronary anatomy. Standard tomographic planes are short-axis, vertical long-axis (equivalent to echocardiographic two-chamber plane), and horizontal long-axis (equivalent to echocardiographic four-chamber plane). In each image plane, the LV is divided into three segments from base to apex—basal, mid-cavity, and apical—to correspond to proximal, middle, and apical lesions of the coronary arteries (Fig. 8-3). In a short-axis view, both at the basal (mitral valve) and mid-cavity (or papillary muscle) levels, the ventricle is divided clockwise, beginning with the interventricular groove, into six segments: the anterior wall, the anterolateral wall, the posterior (or inferolateral) wall, the inferior wall, the inferior septal wall, and the anterior septal wall. The apical region is divided into four segments because of the normal tapering of the ventricle toward the apex: anterior, lateral, inferior, and septal, with an additional segment for the tip of the apex. This results in a total of 17 myocardial segments. The location of wall motion abnormalities can be reported in two-dimensional (2D) or three-dimensional (3D) formats, descriptively using colors to signify wall motion abnormalities or using more quantitative display formats.
Figure 8–3 Segmental analysis of LV endocardial motion and wall thickening.
As shown on the schematics, the LV is divided into three levels from base to apex with wall segment nomenclature as shown. The apex segments are usually visualized from apical four-chamber, apical two-chamber, and apical three-chamber views. The apical cap can only be appreciated on contrast studies in some patients. (From Lang RM, Bierig M, Devereux RB, et al: Recommendations for chamber quantification: A report from the American Society of Echocardiography’s Guidelines and Standards Committee and the Chamber Quantification Writing Group, developed in conjunction with the European Association of Echocardiography, a branch of the European Society of Cardiology. J Am Soc Echocardiogr 18:1440-1463, 2005.)
Evaluation of Left Ventricular Wall Motion
Regional systolic function for each segment of the LV can be assessed on TTE imaging by combining data from multiple image planes or using 3D echocardiography to obtain multiple simultaneous 2D views or a 3D volumetric LV image set. With 2D imaging, standard views for evaluation of wall motion are (Fig. 8-4):
Figure 8–4 Example of the standard image planes used for stress echocardiography.
Images are acquired in a digital cine-loop format at each stress stage and then re-sorted to show the baseline and peak stress images side by side for each view. Images are gated to show only systole so that endocardial motion and wall thickening appear to occur in the same time frame, even though there is a substantial difference in heart rate between baseline and peak stress. Image depth is adjusted to show only the LV. A-long, apical long-axis; A2C, apical two-chamber; A4C, apical four-chamber; SAX, parasternal short-axis.
In the parasternal long-axis view, the basal and mid-ventricular segments of the anterior septum and posterior LV walls are seen. In the parasternal short-axis view, circumferential images of the LV at the base and mid-ventricular levels are obtained. Note that if the transducer is angulated toward the apex from a fixed parasternal position, progressively more apical segments of the posterior wall are imaged while the same segment of the septum is included in the ultrasound image plane. A more parallel alignment between image planes may be obtained by moving the transducer apically to obtain short-axis, mid-cavity, and (sometimes) apical views of the LV (Fig. 8-5) or by using 3D imaging. The apical segments rarely are adequately visualized from the parasternal window or on transesophageal (TEE) images.
Figure 8–5 Effect of transducer angulation on LV imaging.
Angulation of the transducer from a fixed parasternal position results in short-axis views that intersect similar segments of the septum but progressively more apical segments of the posterior wall. By moving the transducer apically, more parallel image planes can be obtained. Ao, aorta.
From the apical window, evaluation of LV wall motion is performed in four-chamber, two-chamber, and long-axis views. Detailed evaluation of the extent of an abnormal myocardium is possible on 2D imaging by slow rotation of the image plane between the standard views. In the four-chamber view, the inferior septum and anterolateral wall are seen. Anterior angulation to include the aortic valve allows visualization of portions of the anterior septum. In the two-chamber view, the anterior and inferior free walls are seen. Endocardial and epicardial definition of the anterior wall may be difficult because of attenuation by adjacent overlying lung tissue. This problem can be alleviated by careful patient positioning and imaging during held respiration. In the apical long-axis view, the anterior septum and inferolateral (posterior) wall are seen (analogous to the parasternal long-axis view). Care is needed in positioning the transducer at the apex to avoid foreshortening of the ventricle from this approach. Integration of data from parasternal and apical approaches, taking into account image quality in each view, allows assessment of each myocardial segment in at least two views. Evaluation from a subcostal approach also may be helpful. In the subcostal four-chamber view, the inferior septum and anterolateral wall are imaged. In the subcostal short-axis view, the inferior and inferolateral (posterior) walls are nearest the transducer and the anterior and anterolateral walls most distal (Fig. 8-6).
Figure 8–6 Comparison of parasternal, subcostal, and TEE transgastric short-axis views of the LV.
Correct identification of wall segments is facilitated by noting the position of the septum and the papillary muscles.
Real-time 3D multiplane or volumetric imaging from an apical approach has the potential to provide more rapid and complete assessment of wall motion (Fig. 8-7). Simultaneous apical views at set angles of rotation or multiple parallel short-axis views can be generated from the 3D volume set, allowing rapid evaluation of wall motion in multiple myocardial segments on the same cardiac cycles. A limitation of apical volumetric scans is that the endocardium is imaged using the lateral, rather than axial, resolution of the ultrasound beam, which may limit identification of endocardial borders for quantitative analysis. When endocardial definition is suboptimal with either 2D or 3D imaging, opacification of the LV with left-sided contrast is recommended for evaluation or regional function (Fig. 8-8).
Figure 8–7 3D echocardiographic wall motion assessment.
Wall motion can be assessed based on 3D volume acquisition image planes corresponding to four-chamber, two-chamber and short-axis images. Wall motion is then displayed graphically on a cine or still image coded by color on the 3D LV reconstruction (top), as a graph of motion versus time (middle), with each segment shown in a different color or as a target diagram (bottom), or with the apex in the center and the base at the edges of the circle with wall segments as shown in Figure 8-3.
Figure 8–8 Contrast enhancement of LV endocardial borders.
This patient had suboptimal endocardial definition even with harmonic imaging and careful patient positioning. After intravenous injection of a contrast agent into the left side of the heart, opacification of the LV chamber is seen with clear definition of the endocardial border, at rest and with stress in the apical four-chamber view, demonstrating inducible ischemia in the mid and apical inferior septum.
Sequence of Events in Ischemia
In contrast, ischemia is a reversible imbalance in the myocardial oxygen demand-to-supply ratio. Even with substantial coronary artery narrowing, blood flow is adequate for myocardial oxygen demands at rest. However, when the narrowing exceeds approximately 70% of the luminal cross-sectional area, blood flow becomes inadequate to meet increased myocardial oxygen demands with exercise, pharmacologic interventions, or mental stress, resulting in ischemia. When oxygen demand returns to baseline, blood flow again is adequate, ischemia resolves, and wall motion returns to normal. Thus, wall motion at rest is normal in patients with coronary artery disease if there has been no prior myocardial infarction.
The sequence of changes as a region of myocardium becomes ischemic is as follows (Fig. 8-9). The first detectable changes associated with heterogeneity of flow to the LV are biochemical followed by a significant perfusion defect (detectable by radionuclide and magnetic resonance techniques). Next, regional myocardial dysfunction, characterized by both abnormal diastolic function and impaired systolic wall thickening, occurs in rapid succession (within a few cardiac cycles). Ischemic ST-segment depression on ECG and clinical angina are relatively late manifestations of ischemia and are not seen consistently. Echocardiography, by detecting abnormal regional wall motion, provides a useful noninvasive method for evaluating ischemia that is more sensitive than electrocardiography given this sequence of events. Echocardiography differs from radionuclide techniques in that the functional consequences of ischemia, rather than the pattern of myocardial perfusion, are assessed.
Figure 8–9 Schematic diagram of the sequence of events in myocardial ischemia.
The level of stress is shown on the horizontal axis, often estimated by the product of heart rate × blood pressure, with the degree of ischemia shown on the vertical axis. With a 70% coronary artery narrowing (blue), ischemia begins when the stress level results in inadequate coronary blood flow to that region of myocardium. As ischemia increases, the sequence of events is shown. With rest (downward blue arrow), these events reverse unless the duration of ischemia is long enough to cause infarction. The onset and slope of the ischemic response is earlier and steeper with more severe coronary stenosis (as shown for a 90% lesion), and later and less steep with milder coronary disease (as shown for a 50% stenosis). ECG, electrocardiogram.
Evaluation of Global and Regional Ventricular Function
Global LV systolic function can be evaluated either qualitatively or quantitatively in patients with coronary artery disease using the approaches described in Chapter 6. Because the pattern of LV dysfunction typically is not uniform, it is important that qualitative and quantitative evaluation be based on multiple tomographic views or 3D imaging. In patients with coronary artery disease, LV ejection fraction measurement provides essential clinical data because it is a crucial variable in clinical decision making.
Segmental (or regional) LV systolic function most often is evaluated using a semiquantitative scoring system based on 2D or 3D imaging. The endocardial motion for each defined myocardial segment is graded as normal, hypokinetic, akinetic, dyskinetic, or aneurysmal (Table 8-1). Some clinicians prefer to subclassify the degree of hypokinesis as mild, moderate, or severe, but such a subclassification often has significant interobserver and intraobserver variability. Ischemia results both in a decrease in the total amplitude and velocity of endocardial motion and wall thickening and in a delay in the onset of contraction and relaxation. Some centers use a numerical scoring system for wall motion from 1 (normal) to 4 (dyskinetic) for each segment. An overall wall motion score index can be derived by dividing the sum of scores for each segment by the number of segments evaluated:
TABLE 8-1
Qualitative Scale for Assessment of Segmental Wall Motion
Wall Motion Grade | Definition |
Normal | Normal endocardial inward motion and wall thickening in systole |
Hypokinesis | Reduced amplitude (<5 mm) and velocity of endocardial motion and wall thickening in systole. Delay in the onset of contraction and relaxation |
Akinesis | Absence of inward endocardial motion (<2 mm) or wall thickening in systole |
Dyskinesis | Outward motion or “bulging” of the segment in systole, usually associated with thin, scarred myocardium |
Aneurysmal | Diastolic contour abnormality and dyskinesis |
Identification of the endocardial border at end-diastole and end-systole
Evaluation of wall motion for all segments of the myocardium
Knowledge of the degree of variability of normal wall motion
Correction for the effects of LV translation, rotation, and torsion
High temporal resolution for analysis of the onset and velocity of myocardial thickening
Approaches that incorporate 3D reconstruction of the LV may improve data acquisition times and may diminish the effects of cardiac motion that potentially result in imaging different regions of the myocardium in systole versus diastole for a given tomographic plane. Although wall thickening or the timing and velocity of motion may be more sensitive methods for the evaluation of regional ventricular function, most current approaches continue to rely on endocardial motion. Other promising approaches include (1) contrast echocardiography to improve endocardial definition or evaluate myocardial perfusion and (2) tissue Doppler or speckle tracking strain and strain rate imaging (Fig. 8-10) (see Suggested Reading and Chapter 4).
Figure 8–10 3D strain for regional wall motion.
Graphic display of systolic strain in a normal subject (top two panels) and a patient with an anteroapical LV aneurysm (bottom two panels). Global and regional myocardial strain are both severely reduced in the patient with coronary artery disease compared to those of the other patient, and the normal exquisite synchrony of the time-to-peak strain is lost, which typifies patients with heart failure due to ischemic heart disease.AVC, aortic valve closure. (From: St. John Sutton M, Wiegers SE, (eds): Echocardiography in Heart Failure: Practical Echocardiography Series. Philadelphia: Saunders, 2012, Fig. 6-30.)
Myocardial Ischemia
Because echocardiographic wall motion at rest is normal in a patient with significant coronary artery disease and no prior myocardial infarction, imaging during ischemia is needed for diagnosis. Inducing ischemia during echocardiographic imaging is referred to as stress echocardiography. Ischemia can be induced by increasing myocardial oxygen demand either with exercise or by pharmacologic interventions (Table 8-2).
TABLE 8-2
Type of Stress | Advantages | Disadvantages |
Treadmill exercise | Widely available High workload | Imaging post-exercise only |
Upright bicycle | Imaging during exercise | Imaging may be technically difficult. Lower workload |
Supine bicycle | Imaging during exercise | Lower workload Supine position affects exercise physiology. |
Dobutamine + atropine | Continuous imaging Does not require physically active patient | Potential adverse effects of dobutamine Level of stress achieved |
Vasodilator | Continuous imaging Does not require physically active patient | Potential adverse effects of vasodilator agent Induction of relative flow inequality rather than ischemia per se |
Atrial pacing | Continuous imaging Does not require physically active patient | Requires permanent pacer Does not simulate exercise |
Basic Principles of Stress Echocardiography
Stress echocardiography is based on the concept that an increased cardiac workload is needed to elicit signs of physiologic dysfunction in many types of cardiac disease. For example, in patients with coronary artery disease, resting myocardial blood flow is adequate so that myocardial function, seen on echocardiography as wall thickening and endocardial motion, is normal at rest. However, when cardiac workload is increased, the increased oxygen demands of the myocardium cannot be balanced by an increase in flow in the coronary artery, resulting in ischemia with impairment of myocardial thickening and endocardial motion (Fig. 8-11). An increase in cardiac workload typically is achieved by having the patient exercise, either on a supine bicycle or an upright treadmill, or by infusion of a pharmacologic agent, such as dobutamine, that increases heart rate (HR) and blood pressure (BP). In addition to echocardiographic imaging, key elements in interpretation of stress test results include:
Figure 8–11 Concept of stress echocardiography.
This schematic illustrates a patient with a 70% stenosis in the proximal third of the left anterior descending coronary artery (LAD). At rest (left), endocardial motion and wall thickening are normal. After stress (right), either exercise or pharmacologic, the middle and apical segments of the anterior wall become ischemic, showing reduced endocardial wall motion and wall thickening. If the LAD extends around the apex, the apical segment of the posterior wall also will be affected, as shown here. The normal segment of the posterior wall shows compensatory hyperkinesis. Ao, aorta.
The basic principles of image acquisition for stress echocardiography are to use standard image planes, ensure that all myocardial segments are visualized in at least one (and preferably two) views, use comparable views at rest and stress, and record images in a digital cine-loop format with side-by-side display of rest and stress images. The cine-loop format is essential because otherwise the change in heart rate between rest and stress makes interpretation of wall motion difficult.
The sensitivity of stress echocardiography for the detection of coronary disease depends on acquiring stress images at the maximal cardiac workload. With pharmacologic stress testing, this is rarely an issue, because the stress level can be maintained until image acquisition is complete. However, with exercise stress, the workload declines rapidly on cessation of exercise, so images must be acquired as quickly as possible after exercise. Both the time from stopping exercise and the heart rate at the time of image acquisition compared to maximum heart rate are recorded as indicators of workload. Three-dimensional echocardiographic acquisition systems that allow simultaneous real-time imaging in multiple image planes offer the promise of faster acquisition times at peak stress, with the potential for improved diagnostic sensitivity (Fig. 8-12).
Figure 8–12 Multiplane imaging for evaluation of regional function.
Example of one type of display of full volume 3D stress echocardiogram. The lower right hand panel displays nine simultaneous short-axis views from apex (upper left) to base (lower right) of the LV. The distance between each adjacently displayed short-axis image is equal, and positions of these transverse cropping planes are shown as white lines on the coronal and sagittal crops. (From: Otto CM [ed]: The Practice of Clinical Echocardiography, 4th ed. Philadelphia: Saunders, 2012, Fig. 4-20.)
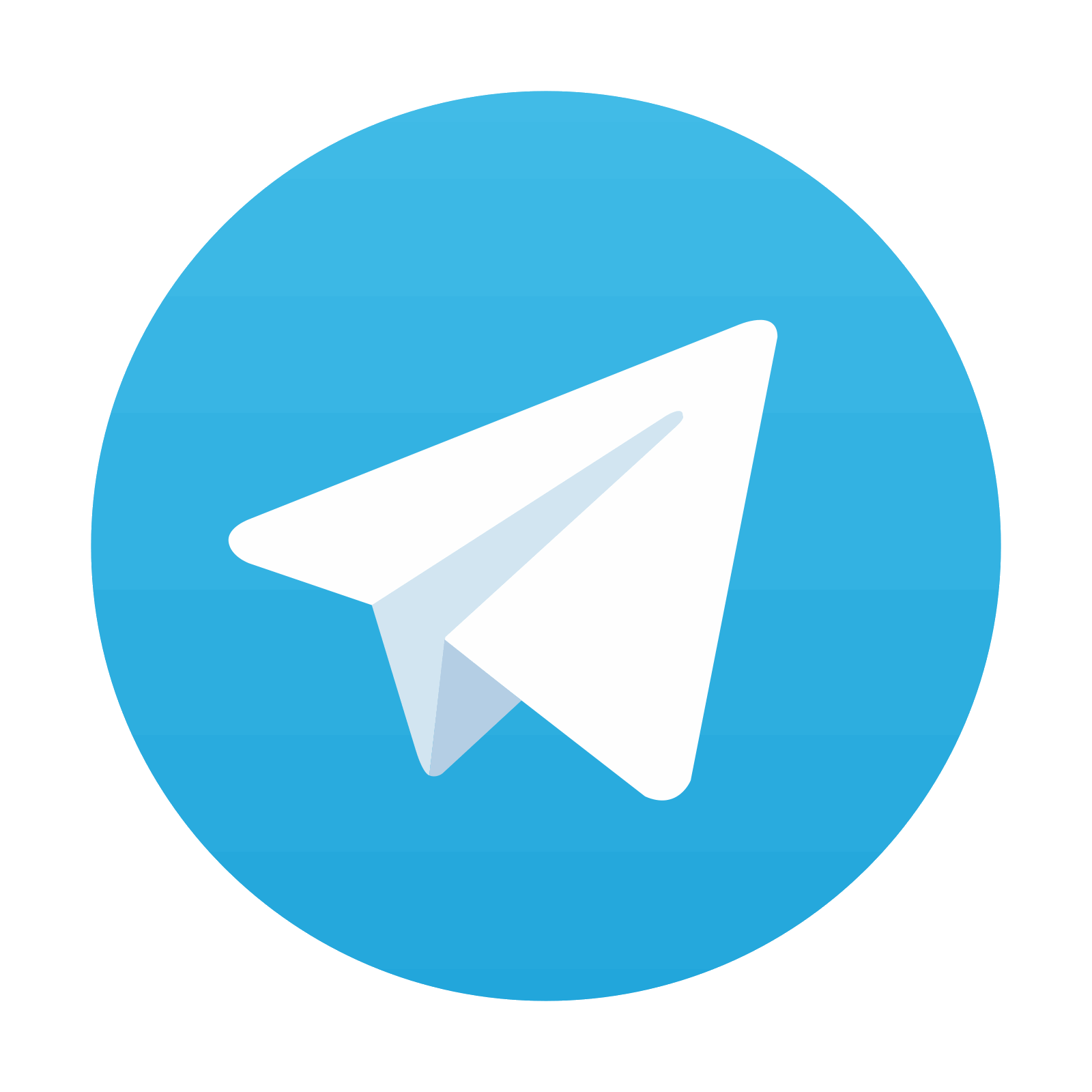
Stay updated, free articles. Join our Telegram channel
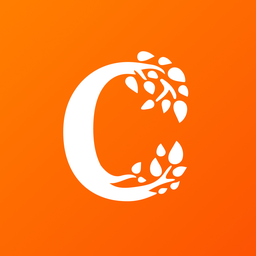
Full access? Get Clinical Tree
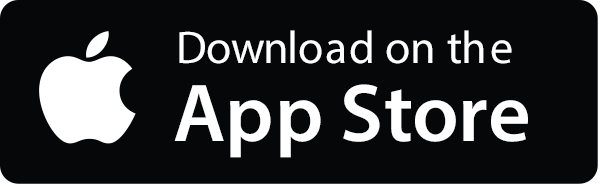
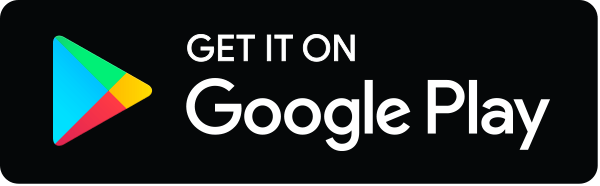