Background
Brown adipose tissue (BAT) consumes glucose when it is activated by cold exposure, allowing its detection in humans by 18 F-fluorodeoxyglucose (FDG) positron emission tomography (PET) with computed tomography (CT). The investigators recently described a novel noninvasive and nonionizing imaging method to assess BAT in mice using contrast-enhanced ultrasound (CEUS). Here, they report the application of this method in healthy humans.
Methods
Thirteen healthy volunteers were recruited. CEUS was performed before and after cold exposure in all subjects using a continuous intravenous infusion of perflutren gas-filled lipid microbubbles and triggered imaging of the supraclavicular space. The first five subjects received microbubbles at a lower infusion rate than the subsequent eight subjects and were analyzed as a separate group. Blood flow was estimated as the product of the plateau ( A ) and the slope (β) of microbubble replenishment curves. All underwent 18 F-FDG PET/CT after cold exposure.
Results
An increase in the acoustic signal was noted in the supraclavicular adipose tissue area with increasing triggering intervals in all subjects, demonstrating the presence of blood flow. The area imaged by CEUS colocalized with BAT, as detected by 18 F-FDG PET/CT. In a cohort of eight subjects with an optimized CEUS protocol, CEUS-derived BAT blood flow increased with cold exposure compared with basal BAT blood flow in warm conditions (median A β = 3.3 AU/s [interquartile range, 0.5–5.7 AU/s] vs 1.25 AU/s [interquartile range, 0.5–2.6 AU/s]; P = .02). Of these eight subjects, five had greater than twofold increases in blood flow after cold exposure; these responders had higher BAT activity measured by 18 F-FDG PET/CT (median maximal standardized uptake value, 2.25 [interquartile range, 1.53–4.57] vs 0.51 [interquartile range, 0.47–0.73]; P = .02).
Conclusions
The present study demonstrates the feasibility of using CEUS as a noninvasive, nonionizing imaging modality in estimating BAT blood flow in young, healthy humans. CEUS may be a useful and scalable tool in the assessment of BAT and BAT-targeted therapies.
Brown adipose tissue (BAT) is a highly vascularized, mitochondria-rich type of adipose tissue characterized by the presence of the uncoupling protein 1 in its mitochondria. The uncoupling protein 1 separates mitochondrial respiration from adenosine triphosphate production and allows protons produced by fatty acid and glucose oxidation to reenter the mitochondrial matrix and generate heat rather than adenosine triphosphate (for a review, see Cannon and Nedergaard ). This process, used mainly for nonshivering thermogenesis, is a highly efficient method of energy conversion. Therefore, the study of BAT has generated high interest as a target for the treatment of disorders such as obesity. BAT is abundant in rodents, and its thermogenic ability has been documented for decades. Until recently, it was assumed that humans possessed functional BAT only in infancy and that it was absent later in life. This assumption has been challenged by 18 F-fluorodeoxyglucose (FDG) studies demonstrating FDG uptake in the supraclavicular space, paravertebral regions, and perivascular tissue surrounding the neck vessels in adults that increases when the subjects are exposed to cold. Tissue analysis from these regions demonstrates the presence of BAT.
The detection of BAT in vivo in humans remains challenging. Positron emission tomography (PET) and, more recently, near-infrared spectroscopy and magnetic resonance imaging, have been used to noninvasively assess BAT. Each noninvasive modality has somewhat unfavorable features, such as accuracy, accessibility, exposure to ionizing radiation, or cost. Presently, 18 F-FDG PET with computed tomography (CT) is the only widely used noninvasive imaging modality that identifies BAT in adult humans. An inexpensive, widely accessible, nonionizing, noninvasive, in vivo technique that would allow the detection of BAT and assess its response to stimuli is very desirable in order to repeatedly assess BAT activation in response to potential therapies or interventions.
BAT is perfused by a rich network of vessels, and activation of BAT is associated with increased blood flow to the tissue. These characteristics may be used to detect BAT and to assess its response to external stimuli. Because perfusion is tightly regulated to respond to the oxygen demand of tissues, one can anticipate that increased BAT metabolism is mirrored by increased BAT perfusion. Therefore, the assessment of BAT perfusion can be used to evaluate the degree of activation of BAT. Contrast enhanced ultrasound (CEUS) is a nonionizing, noninvasive imaging modality that has been used extensively to estimate perfusion, in particular in the myocardium. More recently, investigators have reported the utility of CEUS in determining muscle microvascular responses to physiologic stimuli. We have recently demonstrated that CEUS of BAT blood flow can detect BAT and estimate its mass and activation in mice.
In the present study, we investigated the potential of CEUS to assess BAT in adult humans by imaging blood flow in supraclavicular BAT. To evaluate whether CEUS is able to detect BAT, we colocalized the region where blood flow was detected using CEUS with the region of FDG uptake detected by 18 F-FDG PET/CT. We then investigated whether CEUS could detect changes in BAT blood flow induced by a known stimulus, such as cold exposure. Because BAT blood flow is an important determinant of the delivery of substrates needed for thermogenesis (such as glucose), we also compared the response of BAT blood flow to cold, as assessed by CEUS, to the metabolic activity of BAT after cold exposure, as assessed by FDG uptake on 18 F-FDG PET/CT.
Methods
Protocol
The study was approved by the institutional review boards of the Massachusetts General Hospital and the Joslin Diabetes Center (Boston, MA). Healthy male volunteers between the ages of 18 and 30 years were recruited. Participants fasted for 8 hours before CEUS was performed. A limited transthoracic echocardiographic study was performed on arrival to identify any potential contraindications to intravenous echocardiographic contrast (patent foramen ovale, intracardiac shunt) and to determine stroke volume. Stroke volume was measured as the product of left ventricular outflow tract area and the left ventricular outflow tract time-velocity integral. Cardiac output was measured by multiplying stroke volume by heart rate.
Subjects underwent CEUS after 2 hours of exposure to room temperature (referred to in this study as “warm conditions”), followed by CEUS after 2 hours of cold exposure. Cold exposure was obtained by wearing a cooling vest, with circulating water cooled to 13°C. Imaging was performed in the supine position. Perflutren gas-filled lipid microbubbles (Definity; Lantheus Medical Imaging, North Billerica, MA) were used for contrast enhancement. The initial cohort (group 1; lower infusion rate) of five subjects received 1.3 mL Definity suspended in 30 mL 0.9% saline administered over a 15 min period, with CEUS performed as outlined below. A second cohort (group 2; higher infusion rate) of eight volunteers underwent a modified CEUS protocol, with a higher infusion rate (1.3 mL Definity suspended in 30 mL 0.9% saline, infused over 5 min). In both cohorts, and within 24 hours of CEUS, 18 F-FDG PET/CT was performed after 2 hours of cold exposure.
Ultrasound Protocol
Two-dimensional ultrasound (iE33; Philips Medical Systems, Andover, MA) was performed, using a linear-array broadband transducer with a range of 3 to 9 MHz and a transmit frequency of 3.1 MHz. A multipulse cancelation technique (Power Modulation) was used. Lower powered pulses induce a linear response from the structures imaged, and higher powered pulses induce a linear response from tissue and a nonlinear response from microbubbles. Subtracting one from the other allows detection of the nonlinear behavior that emanates from the microbubbles. The ultrasound probe was positioned in the right supraclavicular fossa, 2 cm superior to the junction of the medial one third and lateral two thirds of the clavicle, to delineate a region of adipose tissue bordered by the lungs inferiorly and posteriorly, the sternocleidomastoid muscle medially and the trapezius muscle laterally ( Figures 1A and 1B ). Color-flow Doppler was used to exclude vascular structures. The focus was set to the middle of the adipose tissue region, which was 1 to 3 cm below the surface of the skin. The gain was optimized so that sparse echoes could be seen from the muscles fibers, and the gain remained unchanged throughout the procedure. Images were recorded at a low mechanical index (MI) setting (MI = 0.25) for 90 sec to ensure that the microbubble concentration reached a steady state. The MI was then adjusted to a higher output (MI = 1.6). The triggered imaging sequence was then started, with pulse intervals of 0.06 (baseline), 0.25, 0.5, 1, 2, 3, 4, 5, 7, 8, and 10 sec. At least three images were obtained for each interval. Blood pressure and heart rate were recorded before, during, and for 15 min after the infusion of contrast. Electrocardiographic monitoring was continuously performed during contrast infusion.

Ultrasound Analysis
The analysis was performed by an observer blinded to the stage of the protocol. A region of interest (ROI) was traced around the adipose tissue, and baseline intensity was measured. The ROI was positioned between the sternocleidomastoid muscle and the trapezius, 1 to 3 cm below the surface of the skin. The extent of the ROI was determined by the amount of adipose tissue present. This ROI was maintained throughout the pulse sequence and in the warm and cold environments for a given volunteer. The mean signal intensity within the ROI was averaged for each pulse interval. The baseline signal intensity was subtracted from each value, and the intensities were plotted on a curve described by the function y = A (1 − e −β t ), where y is intensity at pulse interval t , A is the plateau intensity (in acoustic units [AU]), and β is the rate constant (in sec −1 ), as described previously. Blood flow was estimated as the product of A and β (in AU/sec).
Fluorine-18-FDG Positron Emission Tomographic/Computed Tomographic Protocol
Fluorine-18-FDG PET/CT (Siemens Biograph 64; Siemens Healthcare, Erlangen, Germany) was performed within 24 hours of CEUS. All subjects fasted for 8 hours before arrival in the department. Each participant wore a cooling vest with circulating water at 13°C for 60 min before the intravenous administration of 10 mCi 18 F-FDG and continued to wear it for 60 min after injection. After 60 min of circulation time (2 hours of cold exposure), positron emission tomographic images were acquired in three-dimensional mode starting with noncontrast CT (120 keV, 50 mAs) for attenuation correction. All subjects were imaged in the supine position. Three bed positions were used, with 15 min per bed position.
Fluorine-18-FDG Positron Emission Tomographic/Computed Tomographic Analysis
Fluorine-18-FDG positron emission tomographic/computed tomographic images were analyzed by an investigator blinded to the subject clinical information. Fluorine-18-FDG uptake was measured within the right supraclavicular fossa between the sternocleidomastoid and trapezius muscles, 1 to 3 cm from the skin surface, in an area corresponding to that imaged with CEUS, as described above. Avoiding vascular structures and lymph nodes, an individual volume of interest was placed within the supraclavicular fossa to obtain the maximal standardized uptake value (SUV max ). The standard uptake value is a unitless number that describes the decay-corrected tissue concentration of FDG (in kBq/mL) divided by the injected dose per body weight (in kBq/g). The average venous blood uptake of the superior vena cava was used to derive a target-to-background ratio (TBR) from the SUV max as described previously.
Statistical Analysis
Continuous data are presented as mean ± SD or median and interquartile range (IQR). To determine the intra- and interobserver variability of the method, five BAT blood flow estimations were performed by two blinded readers (A.F. and Q.L.). One reader (A.F.) repeated the measure ≥2 weeks after the first measurement. The differences in measurement between the two readers and between the two readings of one reader are reported as absolute values and percentages. Changes in hemodynamic status induced by cold were compared using Student’s t test. Changes in CEUS parameters were compared using the Wilcoxon signed rank test. Responders were defined as subjects whose BAT blood flow increased in response to cold by twofold or more. The comparison of CEUS responders and nonresponders to BAT FDG uptake (defined by SUV max and TBR) was done using the Wilcoxon rank sum test. Analysis was performed using JMP version 10 (SAS Institute Inc, Cary, NC). P values ≤ .05 were considered to indicate statistical significance.
Results
Population
Thirteen male volunteers (mean age, 24.0 ± 2.4 years; mean body mass index, 23.4 ± 3.5 kg/m 2 ) were enrolled in the study.
Proposed BAT Detection by CEUS
Five subjects underwent CEUS imaging in warm conditions and again after cold exposure (group 1; lower infusion rate). Cold exposure lowered the heart rate and significantly increased blood pressure ( Table 1 ). During cold exposure, a progressive increase in the acoustic intensity was noted in the adipose tissue area, with increasing triggering intervals in all subjects, demonstrating detectable blood flow ( Table 2 ). Sufficient contrast concentration was present in all subjects after cold exposure to allow an exponential curve fitting, therefore allowing an estimation of BAT blood flow. The adipose tissue area visualized on CEUS colocalized with the BAT detected on 18 F-FDG PET/CT ( Figure 2 ). The intraobserver variability of the blood flow measurement was −0.26 ± 16%, and the interobserver variability was −1.78 ± 33%. BAT blood flow in warm conditions could not be reliably detected in group 1, because of insufficient contrast.
Condition | HR (beats/min) | SBP (mm Hg) | DBP (mm Hg) |
---|---|---|---|
Warm | 63 ± 11 | 106.6 ± 5.0 | 64.4 ± 4.1 |
Cold | 58 ± 8 | 128.5 ± 11.5 † | 81.1 ± 6.0 ∗ |
A (AU) | β (sec −1 ) | A β (AU/sec) | |
---|---|---|---|
Cold | 5.07 (2.5–9.3) | 0.36 (0.22–0.50) | 1.59 (1.09–2.47) |
Effect of Cold Exposure on Hemodynamic Parameters and on Blood Flow Estimated Using CEUS
To evaluate whether BAT blood flow increased with BAT stimulation, eight subjects underwent CEUS before and after cold exposure using a higher contrast infusion rate (group 2). Cold exposure induced decreases in heart rate ( P < .001) and cardiac output ( P < .02) and an increase in systolic blood pressure ( P < .02) ( Table 3 ).
Condition | HR (beats/min) | SBP (mm Hg) | DBP (mm Hg) | SV (mL/min) | CO (L/min) |
---|---|---|---|---|---|
Warm | 60 ± 10 | 112.6 ± 10.2 | 67.3 ± 4.0 | 74.5 ± 22 | 4.4 ± 1.2 |
Cold | 50 ± 9 ∗ | 119.0 ± 12.3 ∗ | 73.5 ± 7.2 ∗ | 77.5 ± 21 | 3.8 ± 0.9 † |
The CEUS protocol was modified for group 2 to enhance the detection of BAT blood flow. Using a higher infusion rate (1.3 mL Definity suspended in 30 mL 0.9% saline, infused over 5 min), a plateau in the acoustic intensity was noted 45 ± 10 sec after the initiation of the microbubble infusion in the warm conditions and 43 ± 8 sec after the initiation of the microbubble infusion in the cold conditions ( Figure 3 ). The increase in acoustic intensity with increasing triggering delays was present in all subjects and was sufficient to allow estimation of BAT blood flow both in warm conditions and following cold exposure. All BAT replenishment curves could be fitted to an exponential function ( Figure 4 ). CEUS-estimated BAT blood flow increased significantly following cold exposure (median A β = 3.3 AU/sec [IQR, 0.5–5.7 AU/sec] vs 1.25 AU/sec [IQR, 0.5–2.6 AU/sec]; P = .02) ( Table 4 , Figure 5 ) across the eight subjects. As anticipated, interindividual variation in the response to cold was observed, which allowed us to separate subjects into responders ( n = 5) and nonresponders ( n = 3) on the basis of an arbitrary relative increase in BAT blood flow of twofold or more in responders ( Table 4 ). The responders had higher BAT blood flow, in both warm and cold conditions, than the nonresponders. Blood flow in the trapezius muscle was obtained in all volunteers after cold exposure (median A β = 0.5 AU/sec [IQR, 0.25–0.65 AU/sec]). Blood flow in the trapezius muscle was not obtained in four subjects in warm conditions, because of insufficient contrast.
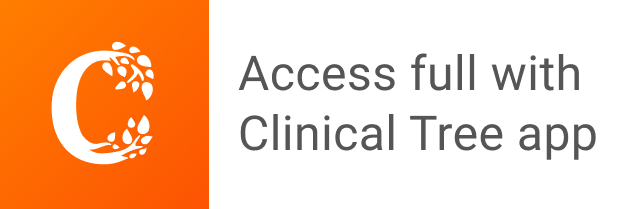