(1)
Project-team INRIA-UPMC-CNRS REO Laboratoire Jacques-Louis Lions, CNRS UMR 7598, Université Pierre et Marie Curie, Place Jussieu 4, 75252 Paris Cedex 05, France
Abstract
Volume 5 aims at providing the fundamental knowledge of the respiratory and vascular tissues that convey and regulate air and blood flows. These serial flows supply oxygen to the body’s tissues and successively remove carbon dioxide produced by the body’s cells. The right cardiac pump drives blood into pulmonary circulation. The pulmonary perfusion must locally match the ventilation. Lung territories oxygenate blood that then returns to the left cardiac pump. The left venticle expels blood into the systemic arterial bed to irrigate all the body’s tissues, including the heart wall and lung parenchyma.
Volume 5 aims at providing the fundamental knowledge of the respiratory and vascular tissues that convey and regulate air and blood flows. These serial flows supply oxygen to the body’s tissues and successively remove carbon dioxide produced by the body’s cells. The right cardiac pump drives blood into pulmonary circulation. The pulmonary perfusion must locally match the ventilation. Lung territories oxygenate blood that then returns to the left cardiac pump. The left venticle expels blood into the systemic arterial bed to irrigate all the body’s tissues, including the heart wall and lung parenchyma.
To raise the surface area of gas exchange in lungs between alveolar air and capillary blood, the design of the tracheobronchial tree relies on branchings of short parent ducts to irrigate and drain all the gas exchange units inside the confined volume of lung parenchyma within the thoracic cage.
Quasi-periodic ventilation is caused by the motion of the thoracic wall, i.e., by cycles of contraction and relaxation of respiratory mucles. The ventilatory pump that limits the external borders of lung parenchymas with their bronchial trees changes the intrathoracic pressure, and hence the volume of deformable bronchioles and alveoli. The end pressure within lung acini enables successively inhalation and exhalation of air from the atmosphere. Rib cage displacements are controlled by the central nervous system.
In blood circulation, the cardiac pump is a component of the blood circuitry. Blood circulates in two serial compartments: pulmonary and systemic circulation. Two closely apposed left and right pumps, each made of two chambers to tackle pressures in the venous and arterial compartments, synchronously expel blood in their respective circuits.
The heart generates starting–stopping-like flows. Arterial unsteady blood flow becomes continuous during the cardiac cycle, but can become bidirectional or even reverse during diastole according to the rheology of the arterial wall.
The vasculature is characterized by a complicated architecture with variable geometry both in space and time. Moreover, the vessel geometry changes over short distances. The vasculature is composed of curved blood vessels with successive branchings to irrigate the body’s tissues.
Adaptation of blood flow to the body’s activity and tissue needs also relies on the central nervous system. In addition, local mechanisms regulate blood flows, using three main mechanisms from large arteries to small arterioles: (1) mechanotransduction-driven postload control; (2) autoregulation; and (3) functional (reactive) hyperemia.
Both air and blood streams are three-dimensional and developing, as they are conveyed in entrance length (Vol. 7 – Chaps. 2. Hemodynamics and 3. Air Transport). Moreover, airways and blood vessels are deformable. Changes in transmural pressure (the pressure difference between the pressure at the wetted surface of the lumen applied by the moving fluid on the pipe wall and the pressure at the external wall side that depends on the activity of neighboring tissues and organs) can also affect the shape of the vessel cross-section, especially when it becomes negative. More generally, the change in cross-section shape can result from taper, possible prints of adjacent organs with more or less progressive constriction and enlargment, and adaptation to branching (transition zone). These changes can induce local three-dimensional blood motions displayed by virtual transverse currents, even if the vessel is considered locally straight.
The magnitude and direction of mechanical stresses applied by air and blood flows on the wetted surface of respiratory epithelia and vascular endothelia as well as within the conduit wall vary during the ventilatory and cardiac cycles. Inside conduit lumens, local changes in the direction of stress components are caused by flow separations and reversal. Flow separation is set by an adverse pressure gradient when inertia forces and blood vorticity are high enough, especially in branching vascular segments. Due to the time-dependent feature of flows, the flow separation region spreads over a variable length during the physiological cycle and can move. The location and variable size of the flow separation region depends on the flow distribution between the branches, which can vary during the physiological cycle. Flow reversal occurs during the diastole of the left ventricle in elastic arteries, such as the aorta, and most of the muscular arteries, such as brachial and femoral arteries (but not in the carotid arteries). Flow reversal can be observed either in a region near the wall, more or less wide with respect to the position of the local center of vessel curvature, or in the entire lumen.
During normal tidal breathing, neurohumoral variations are slight. Breathing influences blood circulation, especially the venous return, by changes in intrathoracic pressure. Because lungs constantly tend to shrink away from the costal pleura, the pressure in the pleural cavity is smaller than that of the atmosphere (negative pressure). Lungs also exert a traction on the pericardium, on arteries in which the blood pressure is high, especially in those of the systemic circulation, and on venae cavae in which the blood pressure is low, thereby deforming intrathoracic veins much more than the intrathoracic arteries. During inspiration, the intrathoracic pressure lowers, thereby reducing right and left auricular preload and right ventricular postload (heightening vascular bore and attenuating flow resistance).1 The cardiac chambers may then receive an amount of blood during inspiration slightly greater than that during expiration. However, the aid given to the right cardiac pump by a low intrathoracic pressure may not significantly influence the left cardiac pump, which works against a much higher outlet pressure range.
In addition, the natural respiratory sinus arrhythmia defines a variation in cardiac frequency during a breathing cycle. The heart beat rate increases during inspiration and decreases during expiration. The cardiac frequency is controlled by the nucleus ambiguus among the centers in the medulla oblongata. The nucleus ambiguus increases parasympathetic nervous system inputs to the heart via the cardioinhibitory vagus nerve. During expiration, neurons in the nucleus ambiguus are activated and the cardiac frequency slows. Conversely, inspiration triggers inhibitory signals to the nucleus ambiguus and consequently the vagus nerve remains in a resting state. In summary, the heart beats more slowly during expiration than inspiration.
Stepwise inspiration and expiration provoke an increase in cardiac frequency followed by a rapid decrease [1620]. The fastest and slowest heart rates are obtained in response to inspiration with respect to expiration. Changes in breathing frequency (from 0.05 to 0.2 Hz) also cause cardiac frequency variations, especially in a respiration frequency range of 0.1 to 0.12 Hz for which heart rate variation is maximal. Heart rate variability with deep breathing serves as a measure of parasympathetic function.
Many factors influence heart rate variability [1621]: (1) age, as the variability decreases with advancing age; (2) body position, as the variability is maximal when the patient is lying supine; (3) respiration frequency, as the variability is maximal when the breathing rate equals 0.08 to 0.1 Hz; and (4) respiration amplitude, as the variability is maximal when the tidal volume is approximately 1.2 l for an average adult.
Both breathing and blood circulation adapt to the body’s needs. Blood contents of oxygen and carbon dioxide primarily control the breathing and cardiac frequencies. Both the body’s ventilation and blood convection are controlled by the autonomic nervous system.
In the central nervous system, four main centers regulate respiration (inspiratory, expiratory, pneumotaxic, and apneustic centers; Table 14.1). Inspiration results from a sudden, ramped increase in motor discharge to the inspiratory muscles. The inspiratory center (dorsal group of respiratory neurons) formed by neurons of the nucleus of tractus solitarius situated in the upper region of the medulla oblongata controls the diaphragm and intercostal muscles responsible for inspiration. These inspiratory muscles are connected to the nervous centers via spinal nerves. Contraction of the diaphragm is triggered by 2 phrenic nerves that emanate from cervical spinal nerves 3 to 5. Eleven pairs of intercostal nerves, the anterior branches (rami anteriores) of the thoracic T1 to T11 spinal nerves regulate the activity of intercostal muscles. Before the end of inhalation, motor discharge declines. Exhalation is usually passive, except at high ventilation rates. At rest, expiration results from the relaxation of the diaphragm and intercostal muscles and lung elastic recoil. A feedback control is ensured by central and peripheral chemoreceptors and mechanoreceptors.
Table 14.1
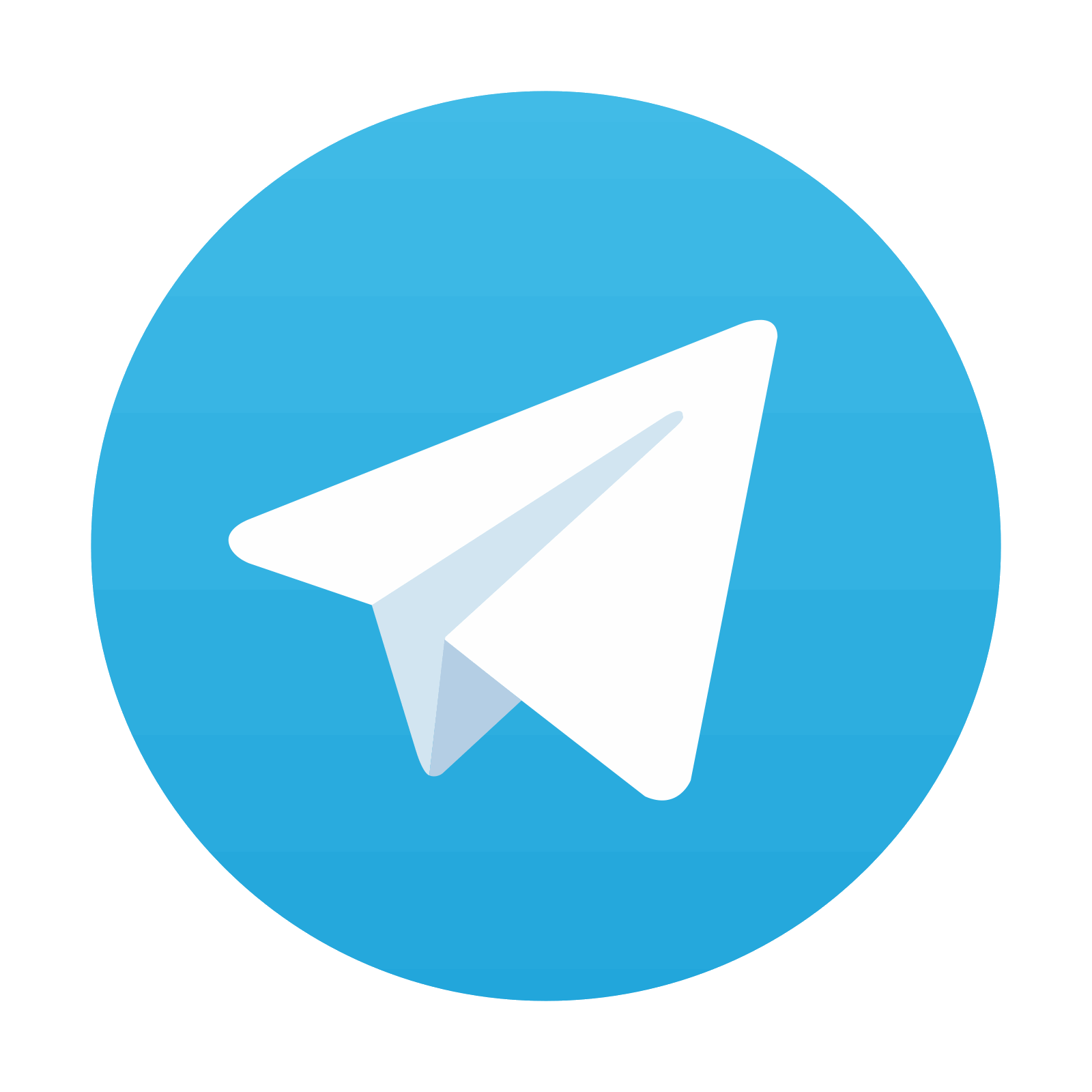
Respiratory centers of the central nervous system.
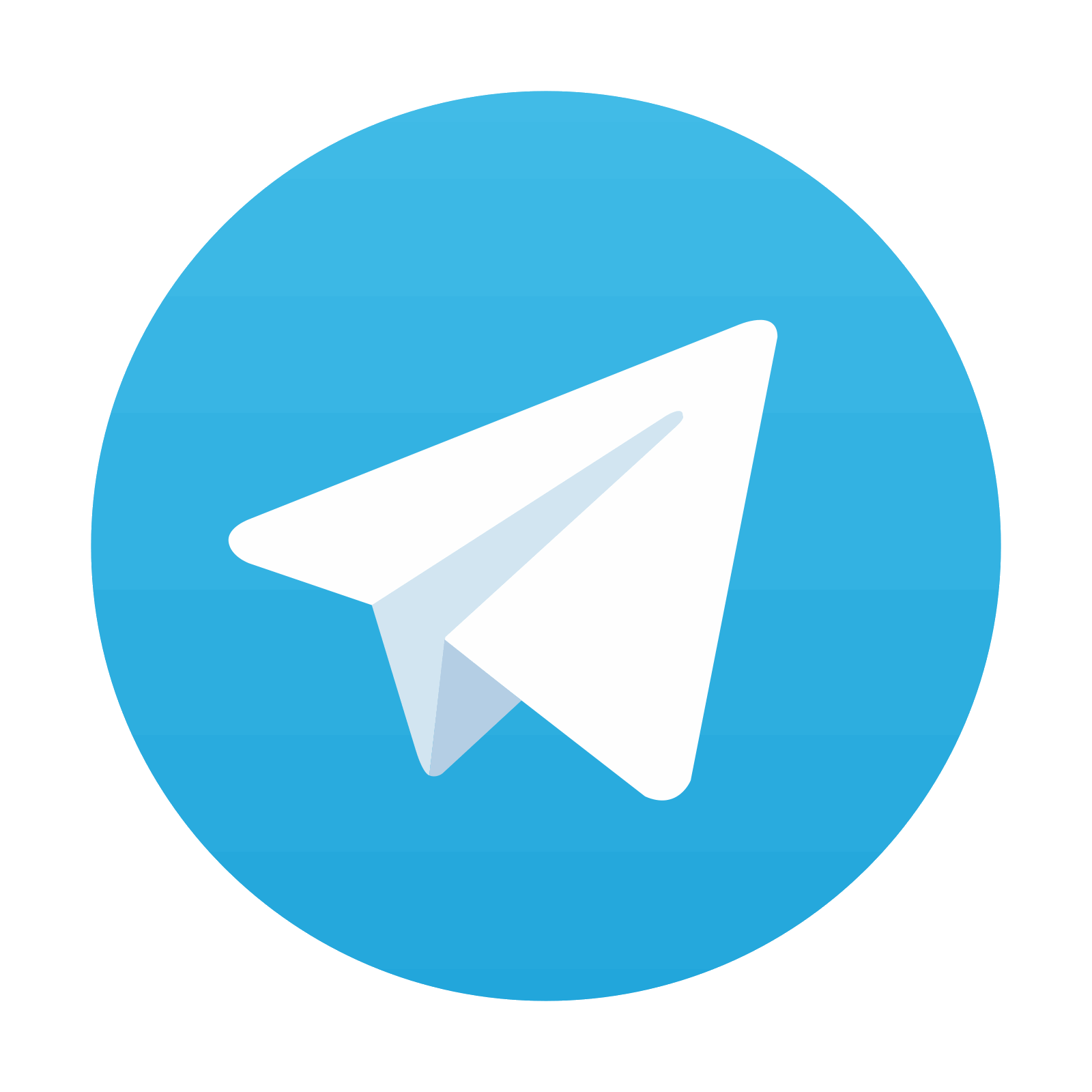
Stay updated, free articles. Join our Telegram channel
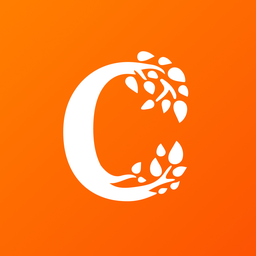
Full access? Get Clinical Tree
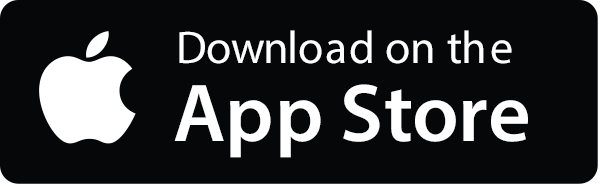
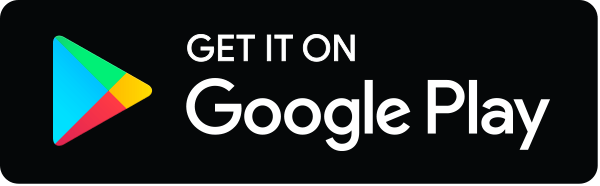
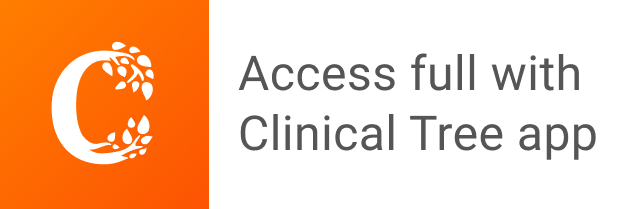