Fig. 8.1
Mechanisms for developing aortic dilatation
8.3 Impedance Mismatch
Characteristic features of aortopathy, including augmented stiffness, aortic dilatation, and subsequent distal narrowing, result in heterogeneity of aortic properties, both in histological feature and aortic diameter. Although energy transmission between two locations of the vessel is maximized if the vascular property is uniform (known as impedance match), part of the blood energy can reflect at any part of the vessel and increase proximal input impedance if a vascular property difference exists [24–26]. In normal circulation, discontinuity of vascular properties in the peripheral arterial system, including relative narrowing and higher stiffness of the peripheral artery compared to that of the aorta, prevents end organs from exposure to high systolic blood energy by reflecting part of the energy. At the same time, it preserves redundant energy in the aortic wall as potential energy so that blood can be delivered to peripheral organs evenly during diastole [27]. In contrast, if central aortic stiffness becomes close to the level of that in peripheral arteries, then more pulsatile energy is delivered to the end organs and can impair their function [28, 29]. This also decreases reserved energy of the aorta, leading to decreased diastolic blood flow. Such discontinuity of vascular properties is called “impedance mismatch,” and this can also accelerate the onset as well as progress of aortopathy by improper augmentation of proximal aortic waveform (Fig. 8.2). In addition to affecting the aorta and systemic circulation, an arising reflection wave in the proximal part of the aorta can be an additional ventricular afterload [21] and can impair ventricular function as well as myocardial energetic efficiency by affecting ventricular–arterial (VA) coupling [30, 31].
8.4 Practical Utility of the Catheter Examination in Aortopathy
Although the shapes of the aorta are easily recognized using MRI or other imaging modalities of three-dimensional images, cardiovascular contrast imaging during catheter examination is two-dimensional and angle dependent, so its utility for morphological assessment is considerably limited in the current era. Meanwhile, having actual pressure waveform is a significant advantage in the assessment of vascular properties in catheter assessment. Catheter examination is commonly performed in patients with congenital heart diseases, so being conversant with such methods would highly support logical decision-making in the clinical setting.
8.4.1 Pulse Wave Velocity
One simple but reliable index for arterial stiffness assessed by catheter examination is pulse wave velocity (PWV). The American Heart Association identified PWV as a noninvasive and reliable marker of aortic stiffness [32]. Although noninvasive PWV is widely used in the clinical setting, PWV is also available during cardiac catheterization. During catheter drawback in the aorta, wave traveling distance is easily obtained by measuring the catheter extraction distance. The time gaps from the ECG R wave to the upstroke of aortic pressure at the starting point and at the end point of extraction represent wave traveling time. Thus, PWV can be calculated as extraction distance divided by wave traveling time [33]. As represented by the Moens–Korteweg equation (Eq. 8.1), PWV increases with the stiffening of the vascular wall, assuming that both vascular wall thickness and vessel area are almost the same.

where E is Young’s modules, h is vascular wall thickness, ρ is blood density, and D is vessel radius diameter. PWV is a predictor of cardiovascular events in heart failure or ischemic heart disease [34, 35]. Higher PWV is reported in patients with Marfan syndrome regardless of aortic dilatation [36], suggesting a possible relationship between PWV and histological change of the tunica media, as well as the predictive value for future aortopathy [37]. As represented in Eq. 8.1, PWV should theoretically decrease with the increase of aortic diameter. Accordingly, the increase of PWV in the dilated aorta further highlights significant stiffening of the aorta. In sharp contrast, PWV in bicuspid aortic valve patients without aortic dilatation was similar to that in tricuspid valve patients [38]. Even though PWV and arterial elastance were similar, aortic wall shear rate was significantly higher in bicuspid aortic valve patients than in tricuspid valve patients [38]. Although prospective observation is required, this result implies a significant contribution of hemodynamics.

(8.1)
We also reported increased aortic stiffness using catheter-based PWV in tetralogy of Fallot (TOF) both before and after anatomical repair compared to control patients [5, 39]. This trend was rather similar to that of Marfan syndrome, and it also implies the possible contribution of genetic factors for the development of aortopathy in TOF. Increased aortic stiffness was also observed in patients with a single ventricular heart, with close correlation with diameter of the ascending aorta [40]. Together, PWV can be a potential predictor for aortopathy, and it may also relate to aortic medial histology. Although those analyses might have been performed even with noninvasive methods [41, 42], catheter assessment allows the consideration of vascular morphological variety, which cannot be assessed with noninvasive methods. More importantly, aortic stiffness in a specific segment is also available if catheter-based PWV is utilized. In TOF patients, ascending aortic stiffness was significantly heightened, whereas that of descending aortic stiffness was not augmented, consistent with the reported distribution of tunica media degradation in similar patient cohorts [3, 43]. Because this method allows us to use PWV of the descending aorta as the internal control, catheter-based PWV is anticipated to be applied to a wide range of cardiovascular conditions.
8.4.2 Assessment of Blood Pressure Waveforms
The other advantage of invasive catheter examination is the availability of a blood pressure waveform at any site of the vessel. Pressure waveform consists of the sum of a forward-traveling pressure wave and a reflected wave (Fig. 8.2) [44, 45]. PWV increases with aortic stiffening, thus allowing the reflection wave to overlap earlier on the subsequent forward pressure wave in the proximal aorta. Because the local blood pressure waveform can be affected by any vascular property change (impedance mismatch) from the distal part of vessel, its morphology identifies how caliber change or stiffness change alters the actual blood pressure waveform. The augmentation index (AI), which is calculated as the augmentation pressure divided by pulse pressure, is the index of the vascular property found by observing the arrival of the wave reflex at a specific site (Fig. 8.3) [46]. Although AI can be affected by the length of the aorta (i.e., patient’s height), heart rate, or cardiac output due to its innate nature, patients with a bicuspid aortic valve were reported to have increased aortic stiffness and high AI, both of which were correlated with aortic dilatation [8], suggesting aortopathy as the source of pressure augmentation. Lee et al. investigated AI using applanation tonometry in bicuspid aortic valve patients and revealed close correlation with E/e′ [47]. Because increased afterload can be a predisposing factor for ventricular stiffening [31], its finding endorses the impact of aortopathy to exert afterload that relates to the reflection wave. Together, the aortic pressure waveform might be a feasible index to determine VA interaction for patients with aortopathy.


Fig. 8.3
Identification of pressure augmentation. AP augmentation pressure, PP pulse pressure. The onset of pressure augmentation by reflection (Ri) was identified by the fourth differentiate of the pressure waveform (Kelly et al.) [73]. (a) Upper panel: Ascending aortic pressure waveform in a patient with a normal aortic arch. The time interval between upstroke of pressure and Ri in the ascending aorta is relatively long, and peak aortic pressure is close to the time of dicrotic notch, allowing higher diastolic pressure. This is suitable for maximizing coronary driving pressure. Lower panel: Descending aortic pressure waveform in a patient with a normal aortic arch. The interval between upstroke and Ri in the descending aorta is shorter than that of the ascending aorta, with systolic pressure higher than that of the ascending aorta. High pressure is suitable for preserving potential energy in the aortic elastic fiber to effectively deliver blood to the peripheral organ. (b) Upper panel: Ascending aortic pressure waveform in a patient who underwent the Norwood procedure. There were two possible inflection points. The first inflection represented the reflection from proximal impedance mismatch, which heightened early systolic pressure. The second reflection wave appeared to be derived from the distal part of the aorta. Lower panel: Descending aortic pressure waveform in a patient who underwent a Norwood procedure. In contrast to Fig. 8.3a, peak aortic pressure was biased to the late systolic phase, which resulted in less systolic pressure. This means impaired preservation of potential energy in the aorta
Pressure assessment using invasive catheterization is increasing its utility in the assessment of aortopathy. The case report published by Murakami et al. suggested aortopathy as a possible source of supra vena cava (SVC) stenosis [48]. Because SVC is a compliant vein and is considered to be durable against compression in general, we need to carefully interpret this case regarding whether surgical scars and postoperative adhesions associated with SVC/aortic cannulation contributed to functional stenosis of SVC pressure. Even with such limitations, catheter insertion is helpful for understanding whether morphological stenosis is hemodynamically problematic, particularly in a lesion where echocardiogram assessment can be inaccurate. Because SVC pressure can affect cerebral circulation [42, 49], assessment of SVC compression and its hemodynamic impact might be of further importance in preventing neurodevelopment or onset of dementia in patients with aortopathy.
8.4.3 Subendocardial Viability Ratio
Due to surgical scars or aortic caliber changes originating from specific operations (i.e., Norwood procedure or Damus–Kaye–Stansel procedure), aortic stiffness or aortic input impedance can be diverse in congenital heart diseases. A similar caliber change is observed in patients with aortopathy even without operation, and the bicuspid aortic valve is often complicated with complex heart diseases. Increased aortic stiffness is a burden for heart failure due to augmented afterload [50], and it also impairs coronary blood flow [51]. This is due to increased ventricular oxygen demand and decreased coronary arterial blood supply, as represented by augmented tension time index (TTI) and decreased diastolic time index (DTI), respectively [52]. Although the subendocardial viability ratio (SEVR) [53] is the simple result of the blood pressure waveform of the ascending aorta, its utility in clinical decision-making is sufficiently validated [52, 54, 55]. The SEVR is not limited to hearts with normal structures. We investigated SEVR in patients after the Norwood procedure, which reconstructs the ascending aorta using the pulmonary trunk and native aorta [54]. In our cohort without aortopulmonary shunts, SEVR was markedly lower than that of control patients with neither significant heart disease nor aortopulmonary shunt in whom all pulmonary blood flow was supplied from the aorta (Fig. 8.4). Accordingly, we concluded that post-Norwood aortas predisposed patients to being more susceptible to coronary ischemia, even without significant coronary stenosis. In this study, aortic impedance mismatch, as was represented by descending aortic relative narrowing compared to that in the ascending aorta, was a significant determinant of SEVR. Furthermore, SEVR was negatively correlated with the renin–angiotensin–aldosterone system and natriuretic peptides (atrial natriuretic peptide [ANP] and brain natriuretic peptide [BNP]), suggesting a promising marker for fibrosis or heart failure. This was further supported by the fact that patient outcome was also associated with SEVR. Our novel finding regarding the role of SEVR emphasizes the importance of guiding proper aortic reconstruction in hypoplastic left heart syndrome, and this might be extrapolated to other congenital heart diseases. The aortic reservoir function in the TOF with aortopathy is expected to be decreased. Although impaired coronary perfusion reserve in patients with decreased aortic distensibility was identified using simultaneous measures of aortic and coronary pressure/flow [56], the possibility that SEVR might also detect similar myocardial blood flow demand–supply imbalances as well as late complications, such as arrhythmias, will be further investigated.


Fig. 8.4
Anatomical features of the subendocardial viability ratio. Mesh area, tension time index (TTI); dotted area, diastolic time index (DTI); filled area, pressure time integral of systemic ventricle. The ratio of DTI to TTI denotes the subendocardial viability ratio (SEVR). SEVR in a patient who underwent the Norwood procedure (RV-PA conduit) was significantly lower than that of controls and even lower than that of patients with pulmonary atresia plus aortopulmonary shunt
8.4.4 Estimation of Aortic Volume Flow
As mentioned, aortic blood flow velocity is closely related to shear stress. Guzzardi et al. investigated aortopathy patients using MR flow mapping and actual aortic wall tissue sampled during surgery, and they elucidated that TGF-β1 and its downstream matrix metalloproteinases are upregulated in the aortic wall exposed to high shear stress but less so in the aortic wall, where less shear stress was observed [12]. Despite the advantages of MRI, which accurately assesses blood flow velocity at a specific location, heart rate variability due to room temperature, the small number of available beats for assessments, and existence of turbulence flow (i.e., pressure recovery in aortic stenosis) are limiting factors for obtaining clinically relevant aortic volume flow. Using cardiac catheterization, the Fick formula also allows us to estimate net aortic blood flow regardless of hemodynamic characteristics or existence of arrhythmias. Although available aortic flow, flow velocity, and shear stress itself cannot be directly estimated using the Fick method, they represent averaged volume loading to the aorta. Our previous data and those of others suggest the significant impact of aortic volume loading on the development of aortic dilatation [5, 6, 13].
The use of simultaneous Doppler flow and pressure wire further allow us to obtain actual blood flow velocity in a beat-by-beat manner with simultaneous measurements of the pressure waveform. Having both the pressure and flow waveforms allows us to factorize pressure/flow waveforms into forward (cardiac contribution) and backward (reflection) flow [44, 57]. The existence of backward flow that overlaps the systolic pressure is attributable to augmented ventricular pulsatile afterload. Accordingly, identifying backward flow and avoiding overlap of the upslope of forward pressure flow by pharmacological intervention will contribute to better management of aortopathy. Westerhof et al. reported the possibility of factorizing the pressure waveform into forward and backward pressure without measuring the flow waveform, but further validation is needed [45].
8.4.5 Cardiac Property
Progression of aortopathy implies afterload increase as well as coronary circulatory insufficiency [9, 21, 51]. Because both affect cardiac performance and geometry [9, 47], assessments of cardiac properties and ventricular–arterial coupling should be considered fundamental constituents of physiology in aortopathy. Venous congestion, which is often accompanied by heart failure, is known to predispose to fibrogenesis and inflammation [58]; therefore, coexistence of cardiac malfunction may accelerate aortic degradation. Using ventricular pressure and simultaneous ventricular volume measurements, the ventricular pressure–volume loop can be constructed either in structurally normal hearts or in those with congenital heart diseases (Fig. 8.5) [59–61]. Load-independent measurements for cardiac contractility (end-systolic pressure–volume relationship [ESPVR]) and diastolic passive stiffness (end-diastolic pressure–volume relationship [EDPVR]) can be determined, and those data allow us to determine therapeutic targets of hemodynamics to improve cardiac function or metabolism [62]. The ratio of end-systolic elastance (Ees) to arterial elastance (Ea) provides VA coupling (Ees/Ea), which is the measure of cardiac performance in relation to afterload [63]. Both ESPVR and coupling ratio (Ees/Ea) are associated with ventricular oxygen consumption in an experimental model [30]. As discussed in the previous section, myocardial circulation in the aortopathy might be impaired, so an energy-preserving strategy might be needed in cases in which unfavorable ventricular energetics is identified. Although aortopathy and ventricular stiffening appear to relate to ventricular diastolic properties, as was suggested by the close relationship between E/e′ and AI in the work by Lee et al. [47], they could not verify how relaxation or passive stiffness relates to arterial property. In addition, the utility of E/e′ was not fully validated in congenital heart diseases [64]; accordingly, more accurate and validated assessments of ventricular diastolic function further support the use of catheter assessment. Accumulating evidence suggests that the coronary microvasculature might be responsible for ventricular diastolic stiffness [65, 66]. In addition to innate cardiac function, external force derived from the pericardium or right ventricle can affect left ventricular preload and stroke volume, which can also be identified by the pressure–volume relationship during right ventricular unloading [67]. While high ventricular diastolic pressure derived from right ventricular dilatation or ventricular stiffening can hinder coronary circulation by decreasing the aorto-ventricular pressure gradient in the diastole, an increase of the RV load demands more coronary flow [68], causing a coronary supply–demand imbalance. Together, PV loops allow us to identify the issues predisposing to cardiac dysfunction coupled with aortopathy. Ventricular mechanics in patients with aortopathy is not well recognized, particularly when there is additional ventricular load. Further investigation is warranted in this field.


Fig. 8.5
Pressure–volume relationship of the ventricle. Ea effective arterial elastance, Ees end-systolic elastance, EDPVR end-diastolic pressure–volume relationship, EDV end-diastolic volume, SV stroke volume. (a) Pressure–volume relationship. Each loop represents the ventricular pressure–volume relationship during volume reduction (IVC occlusion), and the width of each loop represents stroke volume (SV). Ventricular pressure and volume at end systole construct a straight slope, and the end-systolic pressure–volume relationship (ESPVR) and its slope are called the end-systolic elastance (Ees), a load-independent measurement of ventricular contractility. Effective arterial elastance (Ea), a measurement of ventricular afterload, is calculated as the end-systolic pressure divided by the SV. The ventricular diastolic property is characterized by the slope of the pressure–volume relationship of the end diastole (end-diastolic pressure–volume relationship [EDPVR]). (b) Increase of afterload. Aortic stiffening augments the ventricular pulsatile load. Stroke volume declines with the increase of afterload (Red). Even without suppression of Ees, the increase of Ea reduces the Ees/Ea ratio; therefore, ventricular efficiency declines and cardiac energetic consumption increases (pressure–volume area↑). (c) Impaired cardiac contractility. Due to aortopathy and aortic stiffness, coronary perfusion can be suppressed; therefore, the decline of contractility (Ees↓) and the decline of SV can occur
8.4.6 Possible Role of Impedance Analysis
Although PWV and aortic waveforms (AI) are simple but reliable indexes of aortic properties and impedance mismatch, they are relatively sensitive to blood pressure, aortic caliber change, and heart rate. Plenty of evidence that relates aortopathy to its fundamental constituents of focal aortic stiffening is available using PWV or AI; therefore, the additive role of relatively load-independent measurements of vascular properties, such as input impedance, may not be particularly needed in clinical practice. However, advances in noninvasive technology for measuring pressure and flow waveforms in the ascending aorta may expand the utility of input impedance for the management or better understanding of aortopathy.
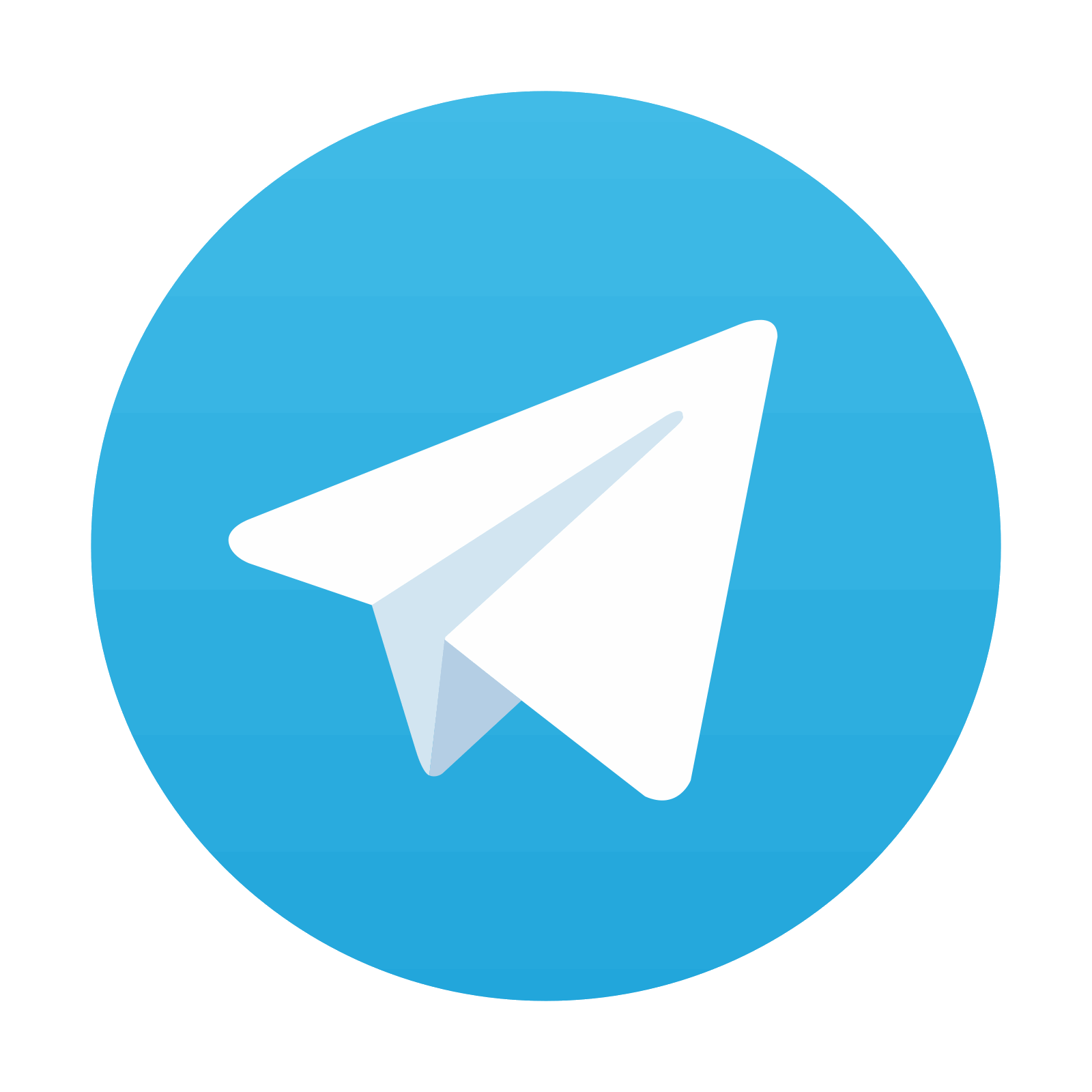
Stay updated, free articles. Join our Telegram channel
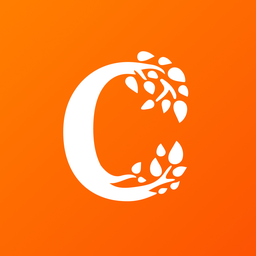
Full access? Get Clinical Tree
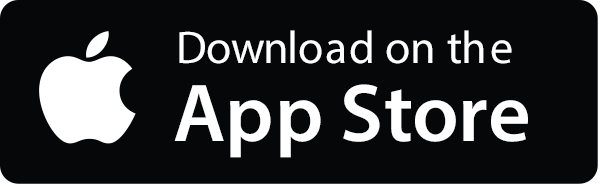
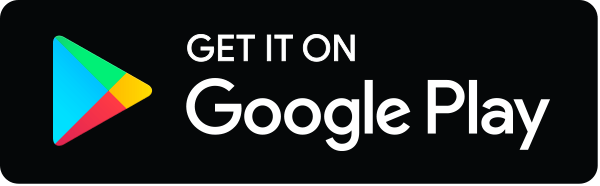