Although the biventricular nature of the disease has been confirmed by morphologic studies, information on right ventricular (RV) function in hypertrophic cardiomyopathy (HC) is lacking. The aim of the study was to hemodynamically characterize RV performance in HC versus idiopathic dilated cardiomyopathy (IDC) during exercise. The hemodynamic data of 63 patients with HC who underwent hemodynamic exercise testing with thermodilution-derived assessment of RV ejection fraction were analyzed. The results were compared to a healthy control group (n = 20) and to patients with IDC (n = 86). The baseline RV ejection fraction was increased in the patients with HC compared to those with IDC (39 ± 10% vs 33 ± 12%; p = 0.002), but did not differ compared to controls (42 ± 7% vs 39 ± 10%; p = NS). An increase in end-diastolic volume from rest to exercise contributed to stroke volume augmentation in those with HC (121 ± 38 vs 136 ± 55 ml/m 2 ; p = 0.01) and control subjects (116 ± 34 vs 138 ± 31 ml/m 2 ; p = 0.002) but not in those with IDC (117 ± 47 vs 120 ± 52 ml/m 2 ; p = NS). At peak exercise the RV ejection fraction in those with HC was reduced compared to that in the controls (45 ± 11% vs 59% ± 9%; p <0.001), but it was increased compared to that in those with IDC (45 ± 11% vs 35% ± 11%; p <0.001). In conclusion, the extent of the pulmonary pressure increase was more pronounced in those with HC than in those with IDC, but the degree of functional impairment of the right ventricle was less severe, probably owing to its ability to recruit preload and contractile reserve with exercise.
The combination of cardiac dysfunction with disease of the myocardium defines the term “cardiomyopathy.” Although it has been confirmed that hypertrophic cardiomyopathy (HC) and idiopathic dilated cardiomyopathy (IDC) are structural processes that affect both ventricles, the main focus of functional assessment has traditionally been placed on the left ventricle. Emerging evidence has shown that the right ventricle is far more than a passive conduit or a silent partner to the left ventricle and that it plays a key pathophysiologic role in heart failure of different etiologies. Maintenance of normal right ventricular (RV) function depends on both the intrinsic factors that determine RV contractile performance and extrinsic factors such as loading conditions that determine RV pump performance. In contrast to the left ventricle, which provides a relatively constant output for a wide range of afterloads, the RV output has been shown to decrease with minimal alterations in afterload. Primary systolic and/or diastolic heart failure might have relatively minor hemodynamic consequences on RV afterload at rest. During exercise, however, when left ventricular (LV) failure worsens and passive back-pressure increases, the right ventricle is exposed to a marked increase in afterload. The present analysis compared the RV functional response to exercise in patients with HC versus IDC.
Methods
All patients with HC or IDC who had undergone clinically indicated hemodynamic evaluation during exercise at our institution from 2004 to 2008 were consecutively screened as potential candidates. Swan-Ganz catheterization with exercise was performed as a part of their clinical evaluation to ensure that the exertional symptoms resulted from circulatory dysfunction. Previous studies have demonstrated that exercise-derived hemodynamic variables provide valuable prognostic information in patients with heart failure and that the cardiac output reserve and the extent of pulmonary pressure increase are hardly predictable from examinations with patients at rest. The primary diagnosis of HC was determined by echocardiographic documentation of a hypertrophied, nondilated left ventricle, unassociated with another cardiac or systemic disease capable of producing a similar magnitude of hypertrophy. IDC was diagnosed by the exclusion of other causes of LV dysfunction. Only patients with New York Heart Association class II to III who had undergone examination during stable medical treatment were accepted. Patients were excluded for the following reasons: more than moderate tricuspid or mitral regurgitation at a subsequent echocardiographic examination, coronary artery disease (stenosis diameter >50%), previous septal ablation, bundle branch block, atrial fibrillation, and disorders other than cardiac disease that limited exercise performance. A total of 63 patients with HC and 86 with IDC satisfied these criteria.
The control group consisted of 20 patients with no evidence of cardiac or pulmonary disease. Of these 20 control patients, 9 were asymptomatic family members of patients with idiopathic pulmonary arterial hypertension and 11 had medically unexplained dyspnea or chest discomfort. In none of these patients could an abnormal increase in mean pulmonary artery pressure >30 mm Hg with exercise be proved. Before this examination, comprehensive pulmonary and cardiac examinations had shown no abnormalities. In those patients with unexplained symptoms, coronary artery disease was excluded angiographically. The control subjects were chosen to closely match the age and gender of the patients with cardiomyopathy. All patients provided written, informed consent before right heart catheterization.
Pressure tracing and assessment of RV function was achieved using a 7.5F, modified Swan-Ganz catheter in conjunction with a computerized detection system of RV ejection fraction (EF) and cardiac output (model REF-1 or Vigilance, Edwards Lifesciences, Santa Anna, California). The method was previously validated by comparison with radionuclide angiography during exercise by our group. A 5-minute period at rest preceded supine incremental cycling until exhaustion against a resistance that was increased by 25 W every 5 minutes. At least 3 thermodilution measurements were performed at each stage for calculation of the mean values of cardiac output, RVEF, and RV end-diastolic volume. Brachial arterial systolic and diastolic pressure were measured by sphygmomanometry. The pulmonary artery systolic pressure to RV end-systolic volume ratio was determined as an estimate of the RV end-systolic pressure–volume relation. The mean arterial pressure and pulmonary vascular resistance were calculated using a standard formula. The cardiac power output was calculated from the equation: cardiac output × mean arterial pressure × 2.22 × 10 −3 . Only completed exercise stages were selected for subsequent analysis.
Transthoracic cardiac ultrasonography in combined M-mode, 2-dimensional echocardiography and continuous/pulsed wave Doppler technique was performed using a Toshiba SSH 140 or Siemens Acuson Sequoia C512. M-mode studies were performed according to previously described criteria. Special care was taken to determine tricuspid regurgitation. Color-flow evaluation of the jet was performed in all obtainable views of the right ventricle and atrium. The degree of tricuspid regurgitation was then graded as mild, moderate, or severe according to a previously published algorithm. The severity of mitral regurgitation was quantified from the apical 4-chamber view by measuring the width of the vena contracta at the narrowest portion of the regurgitant jet. The absence of severe mitral regurgitation was assumed if the vena contracta was <0.5 cm. The LVEF at rest was measured in all patients using gated blood pool or cardiac magnetic resonance imaging.
Statistical analysis was performed after the variables were checked for normal distribution using the Kolmogorov-Smirnov test. In the case of a normal distribution, we applied the paired or unpaired t test and the Pearson linear correlation coefficient. If the data did not show a normal distribution, we used the Wilcoxon test and the Spearman rank correlation. The chi-square test was used for comparison of nominal variables between groups. Analyses were performed using the Statistical Package for Social Sciences, for Windows, version 12.0.1 (SPSS, Chicago, Illinois). All data are given in terms of the mean ± SD.
Results
The data on LV geometry clearly distinguished patients with HC and IDC ( Table 1 ). In the patients with HC, exercise was stopped because of dyspnea or fatigue in 58 patients and chest pain in 5. All patients with IDC stopped exercise owing to dyspnea or fatigue. The HC group included 19 patients with relevant LV outflow tract obstruction (baseline gradient of ≥30 mm Hg or provokable Valsalva gradient of ≥50 mm Hg). An exercise-induced abnormal blood pressure response, as defined by the most commonly used criteria, occurred in 9 patients with HC. However, symptomatic hypotension was not observed. The exercise-related changes in hemodynamic parameters and the results of intergroup comparison are listed in Table 2 . In the controls, the RV end-diastolic volume increased and the RV end-systolic volume decreased with exercise. In the patients with HC, the increase in stroke volume was solely accomplished by an increase in RV end-diastolic volume. The RV end-systolic volume did not decrease significantly with exercise. In the patients with IDC, the stroke volume failed to increase from rest to exercise. The RV end-diastolic volume, RV end-systolic volume, and RVEF did not show an exercise-related change. In the controls, the exercise-related decrease in RV end-systolic volume differed significantly from that observed in the patients with HC and IDC. In both patient groups, the RV end-systolic volume failed to decrease with exercise. Because of this different response, the stroke volume and RVEF increment with exercise was greater in the controls than in the patients with HC. In the patients with IDC, the inability to increase the stroke volume resulted in a significant reduction in the peak exercise stroke volume compared to those with HC. With respect to functional capacity, patients with HC had an intermediate position between control subjects and those with IDC. Although the peak exercise cardiac output was significantly impaired in those with HC compared to that in the controls, it was greater than that in the patients with IDC. In IDC, a reduction in peak exercise stroke volume and heart rate contributed to decreased cardiac output during exercise. The reduction in peak cardiac output was paralleled by a decrease in exercise duration.
Variable | Controls (n = 20) | HC (n = 63) | IDC (n = 86) |
---|---|---|---|
Age (years) | 52 ± 18 | 53 ± 14 | 55 ± 13 |
Body weight (kg) | 83 ± 13 | 79 ± 13 | 84 ± 18 |
Body surface area (m 2 ) | 2.0 ± 0.2 | 1.93 ± 0.2 | 1.99 ± 0.2 |
Gender | |||
Male | 17 | 45 | 66 |
Female | 3 | 18 | 20 |
New York Heart Association class | |||
II | — | 42 | 46 |
III | — | 21 | 40 |
LVEF (%) | 61 ± 8 | 74 ± 11 | 31 ± 13 |
LV end-diastolic diameter (cm) | 4.8 ± 0.4 | 4.9 ± 0.5 ⁎ | 6.2 ± 1.2 † |
Posterior wall (cm) | 0.84 ± 0.1 | 1.1 ± 0.1 † | 1.0 ± 0.1 |
Basal septal thickness (cm) | 0.93 ± 0.2 | 2.1 ± 0.8 ⁎ † | 1.1 ± 0.09 † |
β Blockers | — | 70 ⁎ (%) | 87 (%) |
ACE inhibitor/angiotensin II receptor blocker | — | 19 ⁎ (%) | 93 (%) |
Calcium channel blocker | — | 43 ⁎ (%) | — |
Variable | Controls (n = 20) | HC (n = 63) | IDC (n = 86) | |||
---|---|---|---|---|---|---|
At Rest | Exercise | At Rest | Exercise | At Rest | Exercise | |
Heart rate (beats/min) | 74 ± 16 | 128 ± 25 | 75 ± 20 | 123 ± 26 † | 74 ± 18 | 116 ± 21 ⁎ |
Systolic blood pressure (mm Hg) | 118 ± 12 | 173 ± 21 | 129 ± 37 | 170 ± 33 † | 121 ± 18 | 159 ± 20 |
Mean right atrial pressure (mm Hg) | 3.9 ± 1 | 5.4 ± 2 | 5.1 ± 1 | 9.7 ± 4 ⁎ | 5.3 ± 3 ⁎ | 10.7 ± 7 ⁎ |
Pulmonary artery systolic pressure (mm Hg) | 18 ± 6 | 29 ± 8 | 24 ± 5 † | 48 ± 16 ⁎ † | 23 ± 12 ⁎ | 43 ± 17 ⁎ |
Pulmonary capillary wedge pressure (mm Hg) | 7 ± 3 | 10 ± 2 | 9 ± 3 ⁎ | 25 ± 20 ⁎ | 10 ± 8 ⁎ | 22 ± 11 ⁎ |
Pulmonary vascular resistance (dyn × s × cm −5 ) | 92 ± 55 | 61 ± 57 | 89 ± 83 | 112 ± 64 ⁎ | 126 ± 79 ⁎ | 108 ± 64 ⁎ |
RV end-diastolic volume (ml/m 2 ) | 116 ± 34 | 138 ± 31 | 121 ± 38 | 136 ± 55 | 117 ± 47 | 120 ± 52 |
RV end-systolic volume (ml/m 2 ) | 70 ± 27 | 56 ± 16 | 76 ± 32 | 78 ± 47 ⁎ | 82 ± 47 ⁎ | 82 ± 50 ⁎ |
RV stroke volume (ml/m 2 ) | 46 ± 12 | 81 ± 21 | 45 ± 12 † | 58 ± 16 ⁎ † | 35 ± 10 ⁎ | 37 ± 11 ⁎ |
RVEF (%) | 42 ± 7 | 59 ± 9 | 39 ± 10 † | 45 ± 11 ⁎ † | 33 ± 12 ⁎ | 35 ± 11 ⁎ |
RV end-systolic pressure/volume ratio (mm Hg/ml × m 2 ) | 0.32 ± 0.2 | 0.60 ± 0.3 | 0.37 ± 0.2 | 0.81 ± 0.5 ⁎ † | 0.37 ± 0.3 | 0.67 ± 0.4 |
Cardiac output (L/min × m 2 ) | 2.7 ± 0.8 | 8.9 ± 2.4 | 3.0 ± 0.7 | 6.9 ± 1.8 ⁎ † | 2.6 ± 0.7 | 4.3 ± 1.3 ⁎ |
Cardiac power output (W) | 1.1 ± 0.5 | 4.4 ± 1.2 | 1.2 ± 0.4 | 3.4 ± 1.2 ⁎ † | 1.1 ± 0.5 | 2.1 ± 0.8 ⁎ |
Change in RV end-systolic volume (ml/m 2 ) | −14 ± 24 | 3.1 ± 39 ⁎ | 0.2 ± 37 ⁎ | |||
Change in RV end-diastolic volume (ml/m 2 ) | 21 ± 25 | 16 ± 47 † | 2.1 ± 37 ⁎ | |||
Change in RV stroke volume (ml/m 2 ) | 35 ± 19 | 13 ± 12 ⁎ † | 2.3 ± 9 ⁎ | |||
Exercise duration (minutes) | 27 ± 6 | 16 ± 7 ⁎ | 15 ± 5 ⁎ |
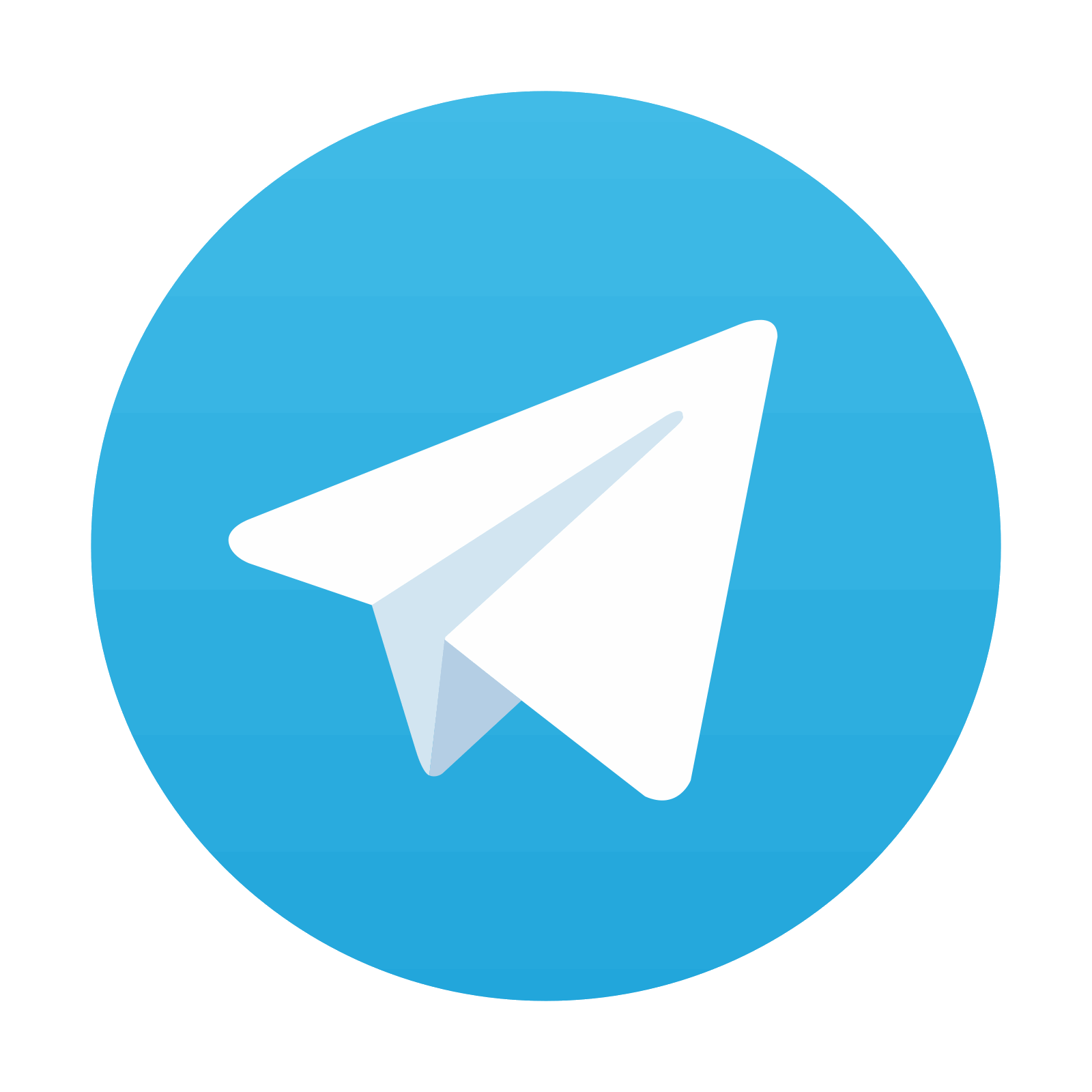
Stay updated, free articles. Join our Telegram channel
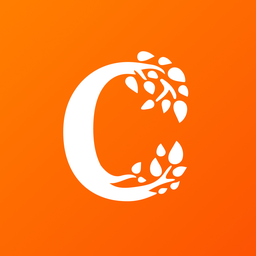
Full access? Get Clinical Tree
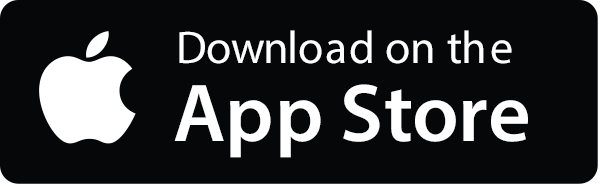
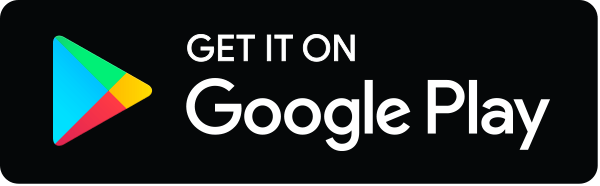
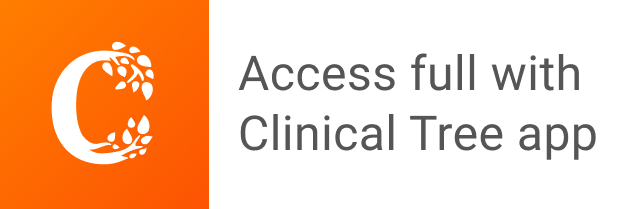