Abstract
This chapter will review the process of normal coagulation in infants and children, examine the various tests of the clotting mechanism, review the alterations of hemostasis in congenital heart disease, consider the impact of cardiopulmonary bypass, extracorporeal membrane oxygenation (ECMO) and ventricular assist device (VAD) use on hemostasis, and develop strategies for the diagnosis and management of bleeding and thrombosis after pediatric cardiac surgery.
Key Words
coagulation, bleeding, thrombosis, cardiopulmonary bypass, ECMO, VAD, hemostasis, fibrinolysis, thromboelastography, platelet dysfunction
In 1950 Bahnson and Ziegler first observed a hemorrhagic diathesis in patients with congenital heart disease (CHD). In fact, hemorrhage was the fourth leading cause of mortality in the first group of patients to undergo the Blalock-Taussig shunt. In his classic article, “A Hemorrhagic Disorder Occurring in Patients With Cyanotic Congenital Heart Disease,” Hartmann noted that 18 patients bled to death after this surgery. He noted that these patients had thrombocytopenia, abnormal clot retraction, low fibrinogen levels, and prolonged bleeding times and observed an association between the degree of polycythemia and the hemorrhagic diathesis.
Over 60 years of progress in hematology and CHD have confirmed Hartmann’s observations. Despite significant advances in the characterization of hemostatic abnormalities in CHD, the precise nature of the defect remains incompletely understood due to its multifactorial nature and individual variation. Preoperative hematologic screening has improved, yet perioperative hemorrhage still complicates approximately 5% of pediatric open-heart surgery, and thrombosis is now recognized as a significant problem often long after the recovery from surgery is complete.
This chapter will review the process of normal coagulation in infants and children, examine the various tests of the clotting mechanism, review the alterations of hemostasis in CHD, consider the impact of cardiopulmonary bypass (CPB) and other mechanical circulatory support devices on hemostasis, and develop strategies for the diagnosis and management of bleeding and thrombosis after pediatric cardiac surgery.
Hemostasis: Cell-Based Coagulation
The classic coagulation cascade model, proposed in 1964 by Macfarlane and Davie and Ratnoff, was accepted for almost 50 years, and although it tremendously improved the understanding of coagulation, we now know this model has some limitations and cannot satisfactorily explain all phenomena related to in vivo hemostasis. The conventional cascade model proposed that coagulation occurs through sequential proteolytic activation of proenzymes by plasma proteases, resulting in the formation of thrombin, which then breaks up fibrinogen to fibrin. This model divided coagulation in an extrinsic pathway (involving components that are usually not found in the intravascular space) and an intrinsic pathway (started by components that exist in the intravascular space), which converge to a common pathway with the activation of factor X. The current cell-based model, on the other hand, proposes that hemostasis requires activated procoagulant substances that remain at the site of the injury and are involved in the formation of platelet and fibrin plugs. The process for clotting is initiated by contact of tissue factor (TF) to the bloodstream. TF is a transmembrane protein that acts as a receptor and cofactor for factor VII (FVII). TF is not constitutively expressed in endothelial cells but is present in the membranes of cells around blood vessels, such as in smooth muscle cells and fibroblasts. Thus TF is exposed to the bloodstream due to damage to the endothelium and surrounding cells or by the activation of endothelial cells or monocytes. As we begin this discussion of the complex process of coagulation, it is important to understand the concept of a cofactor. Cofactors are necessary for the appropriate subsequent reactions to occur, such as TF serving as cofactor for FVII to initiate the clotting process. Calcium is also necessary for this reaction, serves as a cofactor for several other reactions in clotting, and has been referred to as factor IV. Reactions involving cofactors generally require phospholipid surfaces, which become exposed due to injury. This requirement ensures that clot forms only at sites of vascular injury.
The model of cell-based coagulation has been described to have four phases : (1) initiation of coagulation on TF-bearing cells, (2) amplification of the procoagulant signal by thrombin generated on the TF-bearing cell, (3) propagation of thrombin generation on the platelet surface, and (4) termination to prevent pathologic thrombosis. Given the complexity of the coagulation system, it is recommended that you refer to Figs. 24.1 and 24.2 as you read through the phases of coagulation and the role of anticoagulants in this chapter.


Initiation Phase
The initiation phase of coagulation occurs when cells or cellular fragments that express TF on their surface are exposed to blood components at the site of injury. Injury encompasses a number of conditions, including surgery, trauma, and infection. Inflammation releases cytokines, which can also induce cells to express TF in the absence of overt vessel injury, which is why inflammatory conditions are often considered procoagulant states. Regardless of how TF is expressed, once TF is bound to FVII in blood, it quickly activates FVIIa, forming the FVIIa/TF complex, which is responsible for the subsequent activation of small amounts of FIX and FX. The FVIIa/TF complex appears to be essential for initiation of in vivo clotting. FXa can activate factor V (FVa), which is a cofactor for FXa, and together they form a complex called prothrombinase on the surface of cells that express TF. This complex transforms small amounts of prothrombin (factor II) to thrombin ( Fig. 24.1 ). It has been suggested that the initiation phase remains continuously active, with small quantities of activated factors being produced at baseline. Thus small amounts of thrombin are continuously produced outside the vascular space, independent of vascular injury. The coagulation process proceeds to the amplification phase only when there is vascular damage.
Amplification Phase
The amplification phase involves the process of thrombin activation of platelets and cofactors V, VIII, and IX on the surface of platelets. FVIII is actually composed of two parts: (1) factor VIII, which helps IXa activate factor X, and (2) von Willebrand factor (vWF), which binds to a platelet membrane protein (glycoprotein Ib) and promotes platelet adhesion. Due to their large sizes, platelets and FVIII/vWF can pass to the extravascular space only when there is vascular injury. Furthermore, the intact endothelium produces the platelet-inhibiting substances nitric oxide and prostacyclin. When a vessel is injured, platelets leave the vessel and bind to collagen and other components of the extracellular matrix at the site of injury, where they are partially activated and form a platelet plug responsible for primary hemostasis. Small amounts of thrombin produced by cells that express TF as described earlier can then interact with platelets and the FVIII/vWF complex. The FVIII/vWF complex then dissociates, allowing vWF to mediate platelet adhesion and aggregation at the site of injury. This begins the hemostatic process that culminates in the formation of stable fibrin, referred to as secondary hemostasis, which consolidates the initial platelet plug.
Platelet activation by thrombin results in release of the contents of their cytoplasmic granules, including adenosine diphosphate (ADP), serotonin, platelet factor 4 (PF 4 ), catecholamines, and thromboxane A 2 . Upon platelet activation by these released agonists a signalling process is initiated, giving rise to conformational changes within the platelet receptor GPIIb/IIIa. These conformational changes increase the affinity of this receptor for fibrinogen, resulting in platelet bridging and aggregation. Thromboxane A 2 also produces vasoconstriction and causes platelets to further aggregate, recruiting circulating platelets into the primary hemostatic plug formed at the site of vascular injury.
When there is blood vessel wall injury, factor XII is also exposed to collagen or connective tissue in the subendothelium, resulting in its activation. FXIIa catalyzes the conversion of FXI to FXIa. Thrombin also activates FXI (FXIa) on the platelet surface during this phase. FXIa in turn cleaves FIX to FIXa. FIXa then cleaves FX into FXa. However, FIXa requires the cofactor FVIII to complete the reaction. Factor XIIa triggers not only coagulation, but also fibrinolysis, the dissolution of clot. Therefore CPB-induced stimulation of XIIa, for example, causes both a thrombogenic and fibrinolytic response.
Although deficiencies of components of this system, such as FVIII and FIX, in patients result in bleeding, deficiencies of other factors, such as FXII, do not. Ex vivo this system is activated by contact with negatively charged surfaces. Hence factors XI, XII, prekallikrein, and high-molecular-weight kininogen have been termed contact activation factors. There is growing evidence that activation of this system plays a major role in catheter-related thrombi. In addition to activation of thrombin, this system will result in activation of the bradykinin system and thus also participates in vasoregulation.
Propagation Phase
The goal of the propagation phase is to produce large amounts of thrombin and allow bleeding to cease. This phase is characterized by platelet migration to the site of injury and by the production of tenase and prothrombinase complexes on the surface of activated platelets. First, FIX activated during the initiation phase can now bind to FVIIIa on the platelet surface, forming the tenase complex, which activates large amounts of FXa. FXa then associates with FVa bound to the platelet during the amplification phase, resulting in the formation of the prothrombinase complex, which converts large amounts of prothrombin into thrombin. Thrombin is responsible for the cleavage of fibrinogen into fibrin monomers that, in turn, polymerize to consolidate the platelet plug. The formation of insoluble fibrin polymers that are cross-linked by peptide bonds occurs under the influence of factor XIIIa.
Termination Phase
Once a stable fibrin clot is formed at the site of injury, the clotting process must be limited to the injury site to prevent pathologic thrombosis and occlusion of the vessel. Four natural anticoagulants are involved to control the spread of coagulation activation: tissue factor pathway inhibitor (TFPI), protein C, protein S, and antithrombin (AT).
TFPI is a protein secreted by the endothelial cells and platelets that forms a quaternary complex, TF/FVIIa/FXa/TFPI, which inactivates activated factors to limit coagulation. Protein C is a vitamin K–dependent plasma glycoprotein that circulates in an inactive form. However, upon exposure to the endothelial-derived protein thrombomodulin and in the presence of thrombin, protein C is converted to its active form. Once activated, it participates with its cofactor, protein S, to inactivate cofactors V and VIII. Protein S is a vitamin K–dependent plasma protein that enables protein C to more efficiently inhibit binding of Xa to V and to inhibit factor VIII. As a reminder, factor VIII is necessary for the conversion of X to its active form, and FV is necessary to help Xa convert prothrombin to thrombin. AT is a plasma protein that binds to and inactivates thrombin and other serine proteases such as FIXa, FXa, FXIa, and FXIIa, as well as kallikrein and plasmin. Biologic heparan sulphate and medicinal heparin bind to and accelerate the reaction between thrombin and AT, with medicinal heparin accelerating the reaction by up to 10,000-fold. Endothelial cells also produce a variety of glycosaminoglycans (including heparan sulfate), which serve as high-affinity binding sites for AT, which are crucial for quick inactivation of thrombin. There are other physiologic controls that limit the spread of clot. The rapid flow of blood dilutes the effective concentration of activated factors and removes them from sites of injury. Once the activated factors are removed, the hepatic and reticuloendothelial systems promptly and preferentially clear the activated factors from the circulation.
Fibrinolysis
Fibrinolysis involves the dissolution of a stable fibrin clot to allow for restoration of normal blood flow. Once a stable fibrin clot has formed and injured tissues have been repaired, fibrinolysis is initiated through the attraction of plasminogen and tissue plasminogen activator (t-PA) to the lysine residues of fibrin. Plasminogen activators are a heterogeneous group of proteases that are located both within the circulation and in organs such as the lungs, heart, adrenal glands, and ovaries. When it is time to limit the spread of clot, t-PA catalyzes the conversion of plasminogen to plasmin. Plasmin then digests the fibrin clot, which yields fibrin degradation products. Once clot lysis is complete, plasmin enters the circulation, where it is rapidly inactivated by antiplasmin, a serum protein that forms an enzyme-inhibitor complex with plasmin. Thus four reactions counteract fibrinolysis by promoting fibrin stability: (1) factor XIIIa results in cross-linking of fibrin, (2) thrombin-activatable fibrinolysis inhibitor removes lysine residues from fibrin, (3) plasminogen activator inhibitor type 1 (PAI-1) inactivates t-PA and urokinase plasminogen activator (u-PA), and (4) α2-antiplasmin inactivates plasmin.
Two forms of fibrinolysis are worth noting. Primary fibrinolysis is associated with rapid breakdown of clot and is usually due to excess circulating t-PA, as discussed earlier. Secondary fibrinolysis, on the other hand, is a result of hypercoagulability (increased breakdown of clots due to increased formation of clots). Because the causes of primary and secondary fibrinolysis are different, the treatments are also different. In the case of primary fibrinolysis the goal is to treat excessive plasmin activity, which is the consequence of excess circulating t-PA. Administration of an antifibrinolytic agent, with specific or nonspecific antiplasmin activity, is commonly the treatment of choice (see the cardiopulmonary bypass section later) for primary fibrinolysis, whereas anticoagulant therapy is the common treatment for secondary fibrinolysis.
Developmental Changes in Hemostasis
Healthy neonates and infants maintain a balance of procoagulant and anticoagulant states, despite dramatically different levels of the involved factors when compared with older children and adults. Children under 6 months of age have decreased levels of coagulation factors (II, VII, IX, X, XI, and XII) but also decreased levels of other molecules within the antithrombotic pathway (protein C, protein S, and AT). The fibrinolysis pathway also shows age-related changes with diminished plasminogen and tissue plasminogen activator (t-PA) but increased PAI-1. Traditional tests of coagulation function show results consistent with these principles. Studies have shown an inverse relationship between age and prolongation of prothrombin time (PT) and partial thromboplastin time (PTT), with neonates having the greatest prolongation of both tests. Coagulation factor levels typically reach adult values by 6 months to 1 year of age. The presence of low factor levels that might predispose to bleeding is balanced by low levels of circulating inhibitors of coagulation. As a result, infants and young children without heart disease in general are not felt to have an increased propensity toward thrombosis due to the continued balance of procoagulant and anticoagulant factors, which may not be accurately measured by common coagulation studies. However, the delicate balance can easily be disrupted by any number of medical interventions needed in the care of children with cardiac disease, especially in the postintervention period or if the need for mechanical circulatory support arises.
Tests of Coagulation
With the traditional intrinsic and extrinsic coagulation pathways, it made sense that the PT was reflective of the extrinsic pathway (which reflected coagulation outside of the vessel), with the activated partial thromboplastin time (aPTT) reflective of the intrinsic pathway (which reflected coagulation inside the vessel). Given what we now know regarding the interplay between these pathways and other substances leading to the cell-based model, how do we explain what these tests reflect? The PT assesses the levels of procoagulant involved in the initiation phase of coagulation, whereas the aPTT assesses the levels of procoagulant generated during the propagation phase, which are involved in the production of a large amount of thrombin on the surface of activated platelets. No currently available test is able to provide a complete and reliable profile of the hemostatic function.
The normal aPTT is 25 to 35 seconds. The aPTT test will be prolonged when there is less than 30% activity of factors I, II, V, VIII, IX, X, XI, or XII.
The normal PT is 10 to 12 seconds but varies among laboratories. Prolonged PTs are seen when there is less than 30% activity of factors II, V, VII, or X or when there is dysfibrinogenemia or hypofibrinogenemia (factor I). Note that three (factors II, VII, X) of the four vitamin K–dependent clotting factors are evaluated in this test.
What is the significance of vitamin K? The majority of the coagulation factors are synthesized in the liver. Factors II, VII, IX, and X undergo enzymatic transformation in the hepatocyte after synthesis. This transformation involves the addition of a carboxyl (COOH) moiety. Carboxylation is possible only with the help of vitamin K. The COOH addition allows for these factors to bind via a Ca 2+ bridge to phospholipid. Vitamin K–deficient patients produce these factors, but they are nonfunctional. Vitamin K antagonists (VKAs), such as warfarin, exert their anticoagulant effect by inhibiting the carboxylation step in the hepatocyte. Remember that the anticoagulant proteins C and S are also vitamin K dependent.
The international normalized ratio (INR) has been adopted in an attempt to standardize the results of the PT test. INR guides the use of the oral anticoagulant warfarin, which is required in patients at high risk for thrombosis. Typically warfarin is initiated while the patient is still receiving heparin because warfarin will initially decrease protein C levels before achieving therapeutic INR, such that patients may be prothrombotic in the initial stage of warfarin use. Infants require higher doses and take longer to achieve the targeted INR range than older children, such that many choose to use other options for anticoagulation for infants (such as low-molecular-weight heparin [LMWH]). Fontan patients and patients with liver disease require lower Coumadin doses (when it is used), and it is often difficult to determine ideal anticoagulation for such patients.
The activated clotting time (ACT) is a modification of the whole blood clotting time. It is a global measure of anticoagulation and is quickly accomplished at the bedside. The normal range is 80 to 140 seconds. The ACT is performed by automated equipment, and results are provided almost instantaneously. It is used in the operating room as a measure of heparin effect. Heparin activates AT and inhibits FIIa, FIXa, FXa, and FXIa and thus will prolong the ACT. ACT is, however, also affected by hypothermia, thrombocytopenia, and disseminated intravascular coagulation (DIC), to name a few examples in which the result may be prolonged and not be reflective of heparin effect alone.
Tests of Platelet Function
Platelet count is a quantitative measure only, telling us nothing about platelet function. Normal values are 150,000 to 400,000/mm 3 . Platelet counts less than 20,000/mm 3 are usually associated with spontaneous bleeding. Surgical bleeding may occur at counts of 40,000 to 70,000/mm 3 .
Other tests of platelet function available now include the PFA-100, platelet aggregation testing, and thromboelastography (TEG). (Platelet pathways and common antiplatelet therapies are depicted in Fig. 24.2 ). The PFA-100 (platelet function assay) will provide a closure time (CT), which signifies time to formation of a platelet plug. If there is platelet dysfunction, the CT will be increased with epinephrine and/or ADP depending on the disease. For example, PFA-100 may be used to screen for aspirin nonresponsiveness, with CT increased with epinephrine but not ADP. If PFA-100 results are unexpectedly abnormal, formal platelet aggregation testing is then performed in most circumstances.
Light transmission aggregometry (LTA) testing can be used to assess response to different platelet agonists such as thrombin, collagen, arachidonic acid, ADP, epinephrine, and ristocetin cofactor. Born’s PLA is the most widely employed methodology for detecting platelet function disorders and monitoring antiplatelet therapies. It is often the first step in the evaluation of hemorrhagic patients with inherited or acquired platelet dysfunction. The antibiotic ristocetin is another agent used as an agonist in the assessment of platelet function. It facilitates the binding of vWF to the glycoprotein Ib/IX/V complex. For a normal result, both functional vWF and normal glycoprotein Ib/IX/V complex must be present. Hence ristocetin-induced platelet aggregation is considered a test that can detect both von Willebrand disease and platelet dysfunctions. Monitoring antiplatelet therapies (e.g., aspirin) by using LTA has permitted the prediction of major adverse cardiovascular events in adult cardiovascular patients at high risk. Identifying pediatric patients who are nonresponders to antiplatelet agents and are at high risk for thrombosis appears to be important as well because studies have reported 10% to 25% of pediatric cardiac surgical patients are nonresponders to aspirin and aspirin is often the sole anticoagulant used for the Blalock-Taussig shunt and Fontan prophylaxis, for example. In fact, the highest incidence of nonresponsiveness has been reported in patients weighing less than 5 kg given 20.25 mg/d of aspirin.
The VerifyNow system (ITC, Edison, NJ) is a point-of-care test that assesses platelet aggregation in whole blood based on the capacity of activated platelets to bind to fibrinogen. This initial VerifyNow test was used to verify the inhibitory effect of platelet GPIIb/IIIa antagonists (abciximab or eptifibatide) in adult cardiovascular patients undergoing percutaneous coronary intervention and reported as platelet aggregation units (PAUs). Now two more assays, each sensitive to targeted drugs, are available: Aspirin Test with arachidonic acid as agonist, whose results are expressed as aspirin reaction units (ARUs), and PRUTest (sensitive to thienopyridines, such as clopidogrel), whose results are expressed as P2Y12 reaction units (PRUs), by using ADP as agonist.
TEG can also be used to evaluate platelet function with measurement of the maximal amplitude and platelet mapping (see section on TEG later).
Tests of Fibrinolysis
A useful screening test for fibrinolysis is a fibrinogen level combined with D dimer level. If the D dimer level is elevated, fibrinolysis is likely, meaning that the fibrinolytic system is trying to break up a cross-linked clot. D dimer is a specific fibrin degradation product, competing with thrombin to slow down additional clot formation by preventing conversion of fibrinogen to fibrin. Several more specific tests of fibrinolysis are available, including TEG and euglobulin clot lysis time (ECLT). The ECLT is a confirmatory test for fibrinolysis. The euglobulin fraction of plasma is free of inhibitors of fibrinolysis and contains plasminogen, plasminogen activators (primarily t-PA) and fibrinogen. This fraction of plasma is mixed with thrombin, and the time to clot lysis is measured. Normally the ECLT ranges from 90 to 240 minutes. If the ECLT is less than 90 minutes, hyperfibrinolysis is likely. If ECLT is abnormal, it may be useful to undertake specific assays of the components of the fibrinolytic pathway or to perform TEG. TEG often serves to monitor fibrinolysis and is a considerably more rapid test to perform than the ECLT.
Thromboelastography and Thromboelastometry
There has been renewed interest in the use of TEG, first described in 1948, to rapidly assess global in vivo hemostatic function. TEG provides a real-time assessment of viscoelastic clot strength in whole blood. Rotational thromboelastometry (ROTEM) evolved from TEG technology, and both devices generate results of the changes in the viscoelastic strength of a small sample of clotting blood (usually 2 to 3 mL for the test) to which a constant rotational force is applied. These devices allow visual assessment of blood coagulation from clot formation, propagation, stabilization, and clot dissolution ( Figs. 24.3 and 24.4 ). Activation of clot formation can be initiated with both intrinsic (kaolin, ellagic acid) and extrinsic (TF) activators. The two tests use different nomenclature to describe the same parameters. CT (clotting time) for ROTEM and reaction rate (R) for TEG are both defined as the time in minutes it takes for the trace to reach an amplitude of 2 mm. Clot formation time (CFT) and kinetics time (K) are defined as the time necessary for clot amplitude to increase from 2 to 20 mm. Angle (a) is determined by creating a tangent line from the point of clot initiation (CT or R) to the slope of the developing curve. Maximum clot firmness (MCF) for ROTEM and maximum amplitude (MA) for TEG are the peak amplitudes (strength) of the clot. For TEG, Lysis 30 and Lysis 60 (LY30 and LY60) are the percent reductions in the area under the TEG curve, assuming MA remains constant, that occur 30 and 60 minutes after MA is reached. For ROTEM, Lysis Index 30 (LI30) is the percent reduction in MCF that exists when amplitude is measured 30 minutes after CT is detected (see Fig. 24.3 ). Respectively, these parameters measure fibrin formation (R and CT), fibrinogen turnover (K and CFT), speed of clot formation (α-angle), platelet-fibrin interactions (MA and MCF), and fibrinolysis (LY30, LY60, and LI30). Thus R and CT times will be prolonged in the presence of coagulopathy (i.e., factor deficiencies) and shortened in hypercoagulability. The TEG K and α-angle represent cross-linking of fibrin and thus reflect the levels of circulating fibrinogen available for this process to occur. Platelet aggregation is represented in the MA or MCF, with lower values being representative of a potential platelet dysfunction or deficiency with possible fibrinogen deficiency or dysfunction to a lesser degree because fibrinogen is an activator of platelets. The whole blood Thromboelastograph (TEG) Platelet Mapping assay provides a more specific way of detecting the reduction in platelet function, presented as percentage inhibition, by both aspirin and clopidogrel.


TEG and ROTEM may be incorporated into algorithms to diagnose and treat bleeding in high-risk populations such as those undergoing cardiac surgery or suffering from blunt trauma ( Fig. 24.5 ). Some evidence suggests that these algorithms appear to reduce the overall transfusion of erythrocyte and nonerythrocyte blood products compared with empiric therapy, but further study is needed to assess patient outcomes. Additional curves obtained from these tests, such as thrombin generation, may help give insight into thrombus formation, but more work is also needed in this area to determine specific application.

Hemostasis and Coagulation in Congenital Heart Disease
The incidence of abnormalities on preoperative screening tests in CHD patients varies from 20% to 60%. In addition, genetic conditions predisposing to thrombosis (i.e., factor V Leiden, prothrombin gene mutation 20120, and methylene tetrahydrofolate reductase [MTHFR]) have a disproportionally higher prevalence within the CHD population. In general, children with cyanotic heart disease or whose hematocrit values are greater than 60% are most likely to have clinically relevant hemostatic abnormalities. However, acyanotic patients with CHD also manifest dysfunctional coagulation. There is no consistent pattern of abnormality among CHD patients. This wide range and variable presentation are likely due to the heterogeneous population that children with CHD represent. In clinical practice, basic preoperative laboratory assessment includes a hemoglobin and hematocrit determination along with a quantitative platelet count, a PT, and an aPTT. Some centers are beginning to include additional testing, including TEG/ROTEM, factor activity levels, and AT activity in their initial preoperative evaluation for high-risk patients.
Coagulation Factors
Several studies have demonstrated prolongation of the PT and aPTT in CHD. Low levels of factors I (fibrinogen), II, V, VII, VIII, IX, and X have been observed, with elevated levels of factor VIII also being reported, which may be reflective of its role as an acute phase reactant. Quantitative abnormalities are even more pronounced in neonates. Factor levels are approximately 30% to 40% lower in newborns with CHD than in those neonates without structural cardiac abnormalities. One postulated cause for these low levels is impaired hepatic synthesis secondary to liver hypoperfusion, such as following CPB or in hypoplastic left heart syndrome patients with an imbalance of pulmonary-to-systemic blood flow ratio (Q p :Q s ) with inadequate systemic cardiac output as examples. Other proposed mechanisms for decreased production included low cardiac output for a variety of conditions, genetic predisposition, or hepatic synthetic abnormalities, although the latter has not been demonstrated in current studies. Children with cyanotic CHD also often demonstrate delayed maturation of their coagulation system, whereas those with acyanotic heart disease will often demonstrate normal levels by 5 years of age.
The single-ventricle patients are at particularly increased risk of coagulation factor abnormalities. Up to 33% of patients following a Fontan procedure demonstrated thrombotic events, which was initially thought to be multifactorial but in part due to acquired hypercoagulable state from decreased levels of protein C, S, and AT3. However, more recent studies have demonstrated abnormal levels of coagulation factors predating the Fontan surgery. Studies have also demonstrated elevated factor VIII levels in Fontan patients who develop thromboses, which may serve as a predictive tool in the future. Elevated factor VIII levels in adults have been predictive of recurrent deep venous thrombosis (DVT), for example.
Anticoagulant factor abnormalities are also seen with CHD patients, again with increased prevalence among cyanotic children. In cyanotic CHD, hyperviscosity can be the limiting factor in oxygen delivery to tissues because it further decreases flow in small capillaries. This hyperviscosity can occur as a result of increased red cell production and can be further exacerbated by iron deficiency anemia by altering the shape of red cells. Furthermore, AT activity is often significantly lower than in age-matched controls. The utility of replacement in children with documented deficiency remains controversial, but careful repletion may improve coagulation balance or anticoagulation effectiveness. Protein C and S levels are often decreased in CHD patients with a similar exaggeration of the deficiency in both newborns and cyanotic heart disease.
Whatever the exact mechanisms, children at highest risk of coagulation factor abnormalities and thrombosis are those with hematocrit values above 60%, cyanotic defects, left-sided obstructive lesions, and poor cardiac output. Younger age appears to have a variable effect. There are limited data on coagulopathy in adults with CHD, but these patients appear to be at an increased risk compared with their peers. Potential mechanisms include continued abnormal levels of coagulation factors, endothelial dysfunction, mechanical factors, and additive risk from protein-losing enteropathy, pregnancy, or oral contraceptives.
Anatomic considerations include increased blood viscosity within small vessels or shunts. In addition, alterations in blood flow may occur secondary to nonpulsatile flow (such as after bidirectional cavopulmonary anastomosis or Fontan or with mechanical circulatory support) or to vessel or chamber dilation (such as with dilated cardiomyopathy). As a result, children with CHD with extreme alterations in blood flow (stenotic or static) may be at increased risk of thrombosis. However, increased bleeding risk may also occur secondary to increased shear stress, with resultant acquired vWF deficiency (discussed in more detail in ventricular assist device section later).
Platelets
Children with cyanotic CHD demonstrate both quantitative and qualitative platelet defects. Thrombocytopenia occurs in approximately one-third of the children. Even if the absolute platelet count is normal, many of these patients have prolongation of the bleeding time. These findings suggest defects in platelet adhesion and aggregation. Quantitative functional platelet assays have demonstrated elevated levels of beta-thromboglobulin and PF 4 in CHD patients compared with controls. This observation suggests that in CHD, platelets are chronically activated and thus depleted of their vesicle contents. They exist in a functionally refractory state, unable to mount a normal response by aggregating and adhering at sites of vascular injury.
Children with noncyanotic CHD also demonstrate platelet abnormalities. These abnormalities include the loss of the vWF portion of the factor VIII complex. The absence of a portion of vWF leads to bleeding that can be clinically significant. This abnormality is likely due to aberrant flow patterns or altered hemodynamics associated with the specific cardiac lesion, such as in patients with aortic stenosis and in patients with continuous-flow ventricular assist devices (VADs), leading to acquired vWF deficiency. In most affected children studied, the vWF abnormality corrects when the cardiac defect is repaired or the device is removed (see later in VAD section).
Newborns with ductal dependent pulmonary or systemic blood flow receive prostaglandin E infusions preoperatively to maintain ductal patency. These infusions affect platelet function by preventing activation.
Sometimes children receive heparin preoperatively. Heparin-induced thrombocytopenia (HIT) is a rare complication of heparin therapy, occurring with an incidence of 0.5% to 5% in adult patients. The incidence in pediatric patients is presumably less. HIT is an idiosyncratic, antibody-mediated reaction that occurs within 6 to 12 days of heparin administration. The clinical features of HIT are thrombocytopenia (platelet counts typically are around 50,000/mm 3 ; however, values as low as 10,000/mm 3 have been reported) and thrombosis with an increasing heparin requirement. (See HIT section later for additional detail.)
Fibrinolysis
Fibrinolysis may be a contributing factor in the coagulopathy of CHD. Perioperative inhibition of primary fibrinolysis may decrease blood loss during palliative or corrective surgery for CHD through the use of aminocaproic acid or tranexamic acid (discussed in more detail under pharmacologic strategies to treat bleeding).
Anticoagulation Medications
Anticoagulation is frequently used for patients with heart disease, both congenital and acquired, as noted earlier, for prevention or treatment of thrombosis and thromboembolism. Unfractionated heparin (UFH), LMWH, or warfarin is often the anticoagulant of choice in the pediatric population for prevention and treatment of thrombosis. If UFH and LMWH cannot be used due to HIT and if warfarin is not an option due to the lack of oral access or concern for difficulty achieving consistent therapeutic INR, other alternatives such as bivalirudin, argatroban, and fondaparinux can be used. Published literature exists supporting the use of these three latter anticoagulants in pediatric patients.
Unfractionated Heparin and Low-Molecular-Weight Heparin
UFH has been used for the prevention and treatment of thrombosis for several decades. Heparin is mainly obtained from porcine intestine. UFH is a mixture of sulphated glycosaminoglycans of variable lengths and molecular weights and is administered parenterally. Anticoagulant effects and pharmacologic properties vary with the size of the molecules. UFH inactivates several coagulation enzymes, including FIIa (thrombin), IXa, Xa, XIa, and XIIa, by binding to the cofactor AT. Several studies have also shown that heparin possesses various antiinflammatory and immunomodulatory properties. Binding of heparin to different mediators involved in the immune system response (cytokines and chemokines), acute phase proteins, and complement complex proteins may contribute to the antiinflammatory activity of heparin. Use of heparin-treated surfaces in CPB circuits, for example, has been shown to decrease activation of leukocytes and the complement cascades.
LMWHs are derived from UFH by depolymerization. Each LMWH product has a specific molecular weight distribution that determines its anticoagulant activity and duration of action, so one product cannot always be substituted for another. LMWHs in current use globally include enoxaparin, dalteparin, and tinzaparin as examples, with enoxaparin being most commonly used in the United States. They are administered subcutaneously for venous thromboembolism (VTE) prevention and treatment. Like UFH, LMWHs inactivate several coagulation enzymes by binding to AT but have a lower affinity for binding to proteins other than AT and are therefore associated with a predictable dose response and have fewer nonhemorrhagic side effects in patients with normal renal function and without significant inflammation. If these conditions do not exist, LMWHs have improved bioavailability mainly due to reduced reactivity with PF 4 , a release product of activated platelets acting as an antiheparin and as such inhibiting a certain, variable amount of circulating heparin.
Anti-FXa levels are used to measure the effect of UFH and LMWH. Recommended anti-FXa levels in children have been extrapolated from adult studies. In neonates and young infants the PTT may not correspond to anti-FXa levels because of developmental hemostasis. In children or adults with high FVIII or fibrinogen levels (nonspecific acute phase reactants) or in the presence of an antiphospholipid antibody or nonspecific inhibitor, the PTT is not a good reflection of heparin effect. LMWH is a short-chain heparin that does not influence the PTT; therefore the anti-FXa level is the only measure of the effect of LMWH therapy.
Parenteral Direct Thrombin Inhibitors
The most common parenteral direct thrombin inhibitors (DTIs) include argatrobran and bivalirudin. Bivalirudin is cleared by proteolytic mechanisms (80%) and to a lesser degree via renal mechanisms (20%), with argatroban cleared mostly by hepatic mechanisms. DTIs are a useful alternative to heparin for anticoagulation in infants and children. They have been found to be effective for treatment of thrombosis and for anticoagulation during CPB and extracorporeal membrane oxygenation (ECMO) or with VADs. Although they have traditionally been used in patients who were unresponsive to heparin or who developed HIT, they are now being studied as first-line agents. Bivalirudin, unlike heparin, does not require AT to be effective, having the potential to provide more consistent anticoagulation, especially during critical illness. Available literature reports equivalent or lower rates of bleeding or thromboembolic complications. It is more expensive than heparin, but the cost may be offset by reductions in costs associated with heparin use, including anti-factor Xa testing and the need for administration of AT. Studies prospectively evaluating bivalirudin efficacy and safety in the pediatric population are under way.
Warfarin
Warfarin is a vitamin K antagonist (VKA). It is completely absorbed orally and 98% bound to plasma proteins. Warfarin therapy in neonates and infants is associated with significant challenges because of developmental hemostasis with decreased levels of vitamin K–dependent factors in newborns, increased variability in nutritional intake, higher dose requirements, increased incidence of viral infections, and less time in therapeutic range (as high as 50% in some studies). In general, warfarin therapy is not recommended in infants less than 1 year of age unless they have a mechanical valve. A number of drugs are known to interact with warfarin, affecting anticoagulant effect, with some drugs being highly likely to inhibit warfarin, such as carbamazepine, phenobarbital, and rifampin, whereas others increase the risk of bleeding, such as cephalosporins, macrolide antibiotics, acetaminophen, and nonsteroidal antiinflammatory drugs (including aspirin). The mechanism of interference of acetaminophen with warfarin metabolism appears to be through cytochrome P450. If acetaminophen use is necessary, it is recommended to use as low a dose as possible for short duration. Although concomitant use of warfarin and aspirin generally should be avoided, studies in patients at high risk for thromboembolic events (i.e., patients with mechanical heart valves, combination of tissue valves and atrial fibrillation, and VADs) have demonstrated that the increased risk of bleeding with combined warfarin and aspirin therapy is outweighed by the benefit in decreased thromboembolic events.
Genetic polymorphisms may also impact warfarin metabolism. In 2007 the Food and Drug Administration (FDA) approved the Nanosphere Verigene Warfarin Metabolism Nucleic Acid Test capable of detecting variations in the metabolism of two genes, CYP2C9 and VKORC1 . The frequency of these abnormalities varies among individuals and among people of different races and nationalities.
Although there are no well-designed studies evaluating the safety and efficacy of warfarin after the Fontan procedure in children, it may be used for thromboprophylaxis, with typical target INR of 2.0 to 3.0. Lower doses of warfarin are usually needed for Fontan patients, with a typical starting dose of 0.1 mg/kg.
Antiplatelet Medications
Antiplatelet drugs are commonly used in children for both prophylaxis and treatment of thrombosis (see Fig. 24.2 ). Agents that irreversibly inhibit platelet function include aspirin and the thienopyridines clopidogrel, ticlopidine, and prasugrel. Ticagrelor is a direct-acting, reversibly binding, oral P2Y 12 receptor antagonist that exhibits a rapid onset and offset of antiplatelet effect. Other reversible antiplatelet drugs include dipyridamole and cilostazol, and the nonsteroidal antiinflammatory agents also have a transient antiplatelet effect.
Direct Xa Inhibitor
Fondaparinux is an AT-dependent, selective anti-Xa inhibitor with a half-life of 17 to 21 hours in patients with normal renal function, longer if renal impairment exists. In the FondaKIDS trial, dosing of fondaparinux at 0.1 mg/kg subcutaneously once daily in children resulted in pharmacokinetic (PK) profiles comparable to those in adults receiving standard dosing. This study and a subsequent follow-up suggests that fondaparinux can be considered an attractive alternative to LMWH given its once-daily dosing, acceptable safety data, and other favorable properties.
Direct Oral Anticoagulants
Direct oral anticoagulants (DOACs), also known as novel oral anticoagulants (NOACs), are in wide use for adult patients requiring both short-and long-term anticoagulation with increased interest in pediatric use now as well. The increased implementation of DOACs has been due to ease of use, favorable pharmacokinetics with typically short half-lives, decreased drug-drug interactions, and lack of monitoring requirements. Although routine laboratory monitoring is not recommended, it is possible for the DOACs and will be specified later for each drug. Management of patients on DOACs in the perioperative period involves an assessment of thromboembolic event risk while off anticoagulation compared to the relative risk of bleeding if such drug is continued. Based on current adult literature, DOACs are used for treatment and prevention of DVT and PE and for prevention of stroke and embolism in patients with nonvalvular atrial fibrillation and should be avoided in patients who are pregnant and those with mechanical heart valves, rheumatic mitral stenosis, and/or stage V chronic kidney disease. Limited data exist on the use of DOACs in pediatric patients, and none of them currently have FDA-approved pediatric labeling. Off-label use of these DOACs in pediatrics is largely extrapolated from adult dosing guidelines. We will briefly discuss currently available DOACs because we expect their use will increase in the coming years as more data become available for pediatric patients.
Dabigatran etexilate mesylate, known by the brand name Pradaxa, is a DOAC that directly binds to thrombin’s active site, competitively inhibiting both free and clot-bound thrombin, and is therefore an oral DTI. In October 2015 an antidote for dabigatran known as idarucizumab (Praxbind) was approved by the FDA. This is the only DOAC for which there is currently a reversal agent available. PTT or thrombin time will be prolonged with therapeutic dosing. Rivaroxaban (Xarelto) is a DOAC that directly inhibits factor Xa, which is a part of the prothrombinase complex. The most accurate means of measuring rivaroxaban levels in the blood is to perform a calibrated specific anti-Xa assay. Apixaban (Eliquis) is a DOAC that directly and selectively inhibits factor Xa, like rivaroxaban, and therapeutic levels can also be assessed through a specific anti-Xa assay. Edoxaban (Savaysa) also directly inhibits factor Xa, much like rivaroxaban and apixaban. Edoxaban can prolong PTT and the anti-Xa assay, which have both been shown to accurately indicate drug levels within the plasma. Rivaroxaban, apixaban, and edoxaban do not have a drug-specific reversal agent currently available. Current management options for reversal include use of four-factor prothrombin complex concentrate or fresh frozen plasma. There are also reversal agents in development for oral direct anti-Xa inhibitors.
Due to the lack of published clinical trials, it is too early to recommend one of the DOACs as an initial alternative for thrombosis treatment or prevention. More data are needed to determine the appropriate dosing of these agents in different age-groups. To date the available evidence for use of rivaroxaban in this population is from four completed studies, the results of which suggested that in children less than 40 kg of body weight there are significantly reduced serum concentrations, suggesting the need for higher doses. Pharmacokinetic and pharmacodynamic results of apixaban and edoxaban may increase their use in children with study results suggesting different dosing regimens for pediatric patients varying by age, as one would expect.
Thrombosis in Pediatric Cardiac Disease and Use of Anticoagulation
Thromboembolic disorders in pediatric patients have historically been reported to be relatively rare compared with adults. However, during the past 10 years there has been an increase in the incidence of VTE in children. In fact, it was reported that the annual rate of VTE increased by 70% from 2001 to 2007 for pediatric patients, including neonates, infants, children, and adolescents. In the congenital and acquired heart disease population, thrombosis has long been recognized as a potentially life-threatening complication. The American Heart Association (AHA) released a scientific statement, “Prevention and Treatment of Thrombosis in Pediatric and Congenital Heart Disease,” in 2013, citing the prevalence in high-risk groups, including patients with shunt-dependent single ventricles (shunt thrombosis, 8% to 12%; 4% risk of death resulting from shunt failure), postoperative central lines (13% thrombosis in central venous lines [CVLs]), Fontan circulation (17% to 33% incidence of thrombosis after Fontan with 2% to 12% of stroke), arrhythmias, Kawasaki disease with coronary aneurysms, and cardiomyopathy/myocarditis. For most of these conditions there remain a wide variety of preventive and management strategies.
In one study, children with stroke and heart disease had an increased prevalence of one or more prothrombotic abnormalities, including the presence of lipoprotein(a), anticardiolipin antibodies, and protein C deficiency. Thus for evaluation of thrombosis or thromboembolism in pediatric patients, according to the AHA guidelines, it is reasonable to evaluate the following in an initial assessment: levels of protein C, protein S, AT, lipoprotein(a), homocysteine, anticardiolipin antibodies, and lupus anticoagulant; mutations of factor V Leiden; and prothrombin genes. As noted earlier, the incidence of CVL-associated venous thromboembolic events was 13% as documented by venogram in infants and children (158 evaluable patients, all >3 months of age) in patients in the multicenter Prophylaxis of Thromboembolism in Kids Trial (PROTEKT). It is also reported in various studies that in published series, approximately 90% of neonates and 50% of children with venous thrombosis had a CVL with one prospective cohort study demonstrating an incidence of 25%. More specifically, in symptomatic and asymptomatic neonates and children with CHD a prospective cohort study demonstrated an incidence of thrombosis of 25% resulting from CVLs. There is also growing evidence that activation of contact activation factors plays a major role in catheter-related thrombi.
Use of Anticoagulation in Pediatric Heart Disease ( Table 24.1 )
A variety of anticoagulation prophylaxis and treatment regimens exist for a number of cardiac conditions, although there is often a lack of uniformity across institutions, likely related to the lack of prospective pediatric studies. Manlhiot et al. evaluated risk factors associated with significantly increased odds of thrombosis following pediatric cardiac surgery: age greater than 31 days, baseline oxygen saturation less than 85%, previous thrombosis, heart transplantation, use of deep hypothermic circulatory arrest, longer cumulative time with CVLs, and postoperative use of extracorporeal support. Regarding CVL risk in particular, radiographically confirmed asymptomatic CVL-related thromboses (1) may be associated with increased CVL-related sepsis, (2) are the most common source of pulmonary embolism (PE), (3) may result in loss of crucial (and often limited) vascular access, and (4) may have the potential to result in embolic stroke in patients with right-to-left intracardiac shunts. Due to these risks the AHA guidelines state that in infants or children with a CVL who will ultimately require a palliative Fontan procedure or in those with concomitant bacteremia or a hypercoagulable risk factor (increased hematocrit, history of thrombosis, confirmed thrombophilic abnormality), low-dose intravenous heparin may be reasonable until the CVL is removed.
Condition | Anticoagulant/Antithrombotic Prophylaxis | Comments |
---|---|---|
Patients with CVL who will ultimately require a Fontan OR those with concomitant bacteremia OR a hypercoagulable risk factor a | Consider low-dose heparin infusion until CVL removed | Typically initiated once postoperative bleeding controlled or at time of diagnosis of other risk factors |
Shunts: BT or central shunt, Sano shunt | Low-dose heparin infusion if no hypercoagulable risk factors | Typically initiated once postoperative bleeding controlled or at time of diagnosis of other risk factors |
With risk factors a | Consider systemic heparin or DTI if suspected or confirmed infection, known CVL-associated thrombus, stented shunt, or hypercoagulable state (including persistently draining pleural effusions or chylothorax); aspirin as long-term therapy | Consider additional antiplatelet therapy in patients with coronary artery disease or prior stent/shunt thrombus |
Glenn shunt | Aspirin | Usually started within 48 h postoperatively |
With risk factors a | Consider long-term therapy with warfarin or LMWH may be indicated for higher-risk patients (i.e., arrhythmias, ventricular dysfunction, and prolonged immobilization) | |
Fontan shunt With risk factors a | Aspirin Consider long-term therapy with warfarin or LMWH may be indicated for higher-risk patients | Usually started within 48 h postoperatively Consider warfarin for first 3-12 months in high-risk patients Consider addition of warfarin to adolescents/adults |
| Consider aspirin | Usually started within 48 h postoperatively |
Cardiomyopathy with any risk factor a (including shortening fraction ≤10% or ejection fraction ≤25%) | Systemic anticoagulation initially with heparin or DTI or LMWH; transition to warfarin once therapeutic for older patients Consider addition of aspirin | Usually started at diagnosis if no imminent procedures planned and bleeding risk is low |
Patients presenting with shortening fraction ≤20% or ejection fraction ≤45% | Systemic anticoagulation initially with heparin or DTI or LMWH; transition to warfarin once therapeutic for older patients if degree of heart failure persists Consider addition of aspirin | |
Noncoronary endovascular stents (including stented shunts) | Low-dose aspirin for 6 mo | |
With risk factors a | Consider LMWH or warfarin plus aspirin or clopidogrel | |
Stents for higher thrombotic-risk lesions (nonpulsatile flow, previous complete occlusion, thrombophilic abnormality) | Warfarin or LMWH with or without antiplatelet therapy for 3-6 mo after implantation and then continue antiplatelet therapy alone | Consider additional antiplatelet therapy in patients with coronary artery disease or prior stent/shunt thrombus |
Transcatheter ASD closure | Low-dose aspirin for 6 mo Additional anticoagulant may be added for 3-6 mo in older children and adults | |
Transcatheter VSD closure | Low-dose aspirin for at least 6 mo | |
Transcatheter PDA closure | Low-dose aspirin for 6 mo | |
Aortic and/or Mitral Valves | ||
Mechanical aortic valve (e.g., St. Jude, Medtronic, Carbomedics) | Heparin use started 48-72 hrs post-op | |
With no additional thrombotic risk factors | Systemic heparin or DTI until therapeutic warfarin plus aspirin | Goal INR 2-3 |
With risk factors b (plus atrial fibrillation, older-generation mechanical aortic valves) | Systemic heparin or DTI until therapeutic warfarin plus aspirin | Goal INR 2.5-3.5 |
Mechanical aortic valve (On-X) | Systemic heparin or DTI until therapeutic warfarin plus aspirin | Heparin use started 48-72 hrs post-op Goal INR 2-3 for 3 mo, then 1.5-2 |
Mechanical mitral valve (e.g., St. Jude, Medtronic, Carbometrics) | Systemic heparin or DTI until therapeutic warfarin plus aspirin | Goal INR 3 (2.5-3.5) |
Mechanical mitral valve (On-X) | Systemic heparin or DTI until therapeutic warfarin plus aspirin | Goal INR 2.5-3.5 for 3 mo, then 2-2.5 |
Bioprosthetic aortic or mitral valve (use rare in children) | Low-dose aspirin; Consider warfarin for 3 mo | Goal INR 2-3 |
If risk factors present b | Systemic heparin or DTI until therapeutic warfarin plus aspirin | Goal INR 2-3 |
Pulmonary and/or Tricuspid Valves | ||
Bioprosthetic pulmonary valve | Consider aspirin for 3-6 mo | |
Bioprosthetic tricuspid valve | Consider life-long aspirin; consider warfarin for 3-6 mo | Goal INR 2-3 |
If risk factors present b | Systemic heparin or DTI until therapeutic warfarin plus aspirin | Goal INR 2-3 |
a Potential risk factors include (1) atriopulmonary type of Fontan connection, (2) bilateral bidirectional cavopulmonary anastomoses, (3) hypoplastic cardiac chambers with flow stasis, (4) presence of a blind-ended pulmonary artery stump, (5) history of previous thrombosis, (6) protein-losing enteropathy, (7) prolonged pleural effusions/chylothoraces, (8) prolonged immobilization, (9) ventricular dysfunction, (10) arrhythmia, (11) presence of thrombogenic foreign material, (12) atrial-level fenestration, (13) Kawashima connection, (14) polycythemia, and (15) an abnormal thrombophilia profile.
b Potential risk factors for valve replacement: (1) previous thromboembolism, (2) systemic ventricular dysfunction, and (3) hypercoagulable condition.
PE is discussed in more detail in Chapter 74 , but we will briefly mention management here based on AHA guidelines. Infants and children with PE and heart disease who are clinically stable should be treated with anticoagulation according to the guidelines for deep venous thrombosis. In infants and children with PE and heart disease who have life-threatening cardiorespiratory compromise and positive radiographic findings for PE, rapid removal of pulmonary thrombi by thrombolytic therapy, pulmonary embolectomy, or stent placement can be beneficial.
Regarding shunt prophylaxis, low-dose aspirin (1 to 5 mg/kg) is recommended for prevention of long-term polytetrafluoroethylene systemic to pulmonary shunt thrombosis in infants and children. According to AHA guidelines, anti-platelet therapy for long-term antithrombotic therapy is “reasonable” after cavopulmonary anastomosis and “probably indicated” after Fontan procedure, although long-term therapy with warfarin may be indicated after the Fontan procedure for higher-risk patients, such as those with arrhythmias, ventricular dysfunction, and prolonged immobilization. Shunt thrombosis management can be life-threatening and should include immediate systemic anticoagulation with a bolus of intravenous heparin (50 to 100 U/kg) and ongoing heparin infusion, as well as maneuvers to increase Q p with lowering of pulmonary vascular resistance (PVR) and increasing systemic vascular resistance, such as increasing systemic blood pressure (e.g., intravenous phenylephrine or epinephrine) in an effort to improve blood flow through the shunt, and intubation, mechanical ventilation, and neuromuscular blockade to maximize oxygen delivery and to minimize oxygen consumption with consideration of inhaled pulmonary vasodilators to lower PVR. Cardiac catheterization with directed t-PA, surgical exploration, and/or ECMO may be required.
After superior cavopulmonary anastomosis or Fontan palliation, patients are at increased risk of developing pleural effusions, with chylous effusions likely the most concerning. Chylothoraces are associated with an increased risk of thrombosis, as they are associated with the loss of natural anticoagulant proteins, including proteins C and S and AT, the deficiency of which can limit the effectiveness of heparin. Serious thrombotic complications can include pulmonary artery and cerebral sinovenous thrombosis, the latter of which can be associated with stroke. According to the AHA guidelines, anti-platelet therapy for long-term antithrombotic therapy is “reasonable” after cavopulmonary anastomosis and “probably indicated” after Fontan procedure, although long-term therapy with warfarin may be indicated after the Fontan procedure for higher-risk patients, such as those with arrhythmias, ventricular dysfunction, and prolonged immobilization. Given that there is some evidence that the first 3 to 12 months after Fontan procedure may be a higher-risk period, warfarin or LMWH may be indicated, particularly for patients at increased thrombotic risk. Likewise, given that older patients may be at higher risk, escalation or initiation of antithrombotic therapy may be indicated in adolescence or adulthood.
Refractory intraatrial reentrant tachycardia, atrial flutter, and atrial fibrillation are less common in pediatric patients than in adults, but when present may be associated with left atrial thrombus. When a thrombus has been found, the recommendation for adult patients is anticoagulation with a VKA for 4 weeks before cardioversion with a repeat transesophageal echocardiography to confirm resolution of thrombus. In adults, anticoagulation is also recommended for a minimum of 4 weeks after cardioversion. Although there are currently no data in the pediatric age-group on the timing or need for anticoagulation after cardioversion of refractory atrial arrhythmias, it is common pediatric practice to continue postcardioversion anticoagulation.
To prevent coronary thrombosis in coronary aneurysms, such as in patients with Kawasaki disease, aspirin is used, with a second antiplatelet agent added for moderate-size aneurysms (4 to 6 mm). Most commonly, patients with giant aneurysms (≥8 mm), with or without stenoses, are treated with low-dose aspirin, together with warfarin, maintaining an INR of 2.0 to 3.0 or therapeutic LMWH. In patients with giant aneurysms with a recent history of coronary thrombosis, triple therapy may be considered (two antiplatelet agents and LMWH or warfarin).
For cardiomyopathy patients with arrhythmias, previous thrombosis or thromboembolism, thrombophilic conditions, or shortening fraction of 10% or less or ejection fraction of 25% or less, it is “reasonable” to use systemic anticoagulation according to the AHA guidelines. Furthermore, for patients presenting with shortening fraction of 20% or less or ejection fraction of 45% or less, systemic anticoagulation (warfarin or heparin therapy with or without aspirin) for the first three months may also be “reasonable.” Assessment for thrombophilia abnormalities may be undertaken for those patients with thrombus or if VAD use is necessary.
For children undergoing placement of endovascular stents for non-coronary lesions, low-dose aspirin should be used for at least 6 months after implantation according to AHA guidelines.3 In children undergoing stent implantation for higher thrombotic-risk lesions, such as nonpulsatile flow, previous complete occlusion or thrombophilic abnormality, it is “reasonable” to use warfarin or LMWH with or without antiplatelet therapy for 3 to 6 months after implantation and then continue antiplatelet therapy alone according to these same guidelines.
According to the AHA guidelines, for transcatheter atrial septal defect device closure in children, low-dose aspirin should be used for 6 months following implantation, with an additional anticoagulant potentially added to aspirin therapy for 3 to 6 months in older children, while for transcatheter ventricular septal defect closure, low-dose aspirin may be used for at least 6 months after implantation.
Anticoagulation in the first few months after surgical valve replacement has traditionally been used to protect against thrombotic complications, presumably related to suture material and to a device that does not yet have biofilm and endothelialization (a process that usually takes 3 to 6 months).
Based on adult data, the addition of aspirin to VKA therapy in patients with mechanical valves leads to reduction in risk of thromboembolism and mortality when compared to VKA therapy alone (65% observed risk reduction in major systemic embolism or death in the aspirin plus VKA group). The addition of at least 50 to 100 mg/d of aspirin is therefore recommended in the current American College of Cardiology (ACC)/AHA and American College of Chest Physicians guidelines for all patients with mechanical valves, though consideration must be given to an individual patient’s bleeding risk. The ACC/AHA “Guideline for the Management of Patients With Valvular Heart Disease” recommends VKA therapy with a target INR of 2.5 for patients with mechanical aortic valves if no additional risk factors are present (see Table 24.1 ). Additional risk factors are defined as atrial fibrillation, prior thromboembolism, left ventricular dysfunction, or hypercoagulable state. For patients with mechanical mitral valves, older-generation mechanical aortic valves, or risk factors in addition to any type of mechanical aortic valve, a target INR of 3.0 is recommended.
Based on the 2017 focused update on management of patients with valvular heart disease, the AHA/ACC recommends use of a VKA after bioprosthetic valve replacement for aortic and/or mitral bioprosthesis for 3 to 6 months after surgery in patients at low risk for bleeding. In children, however, tissue valves, particularly xenografts, deteriorate rapidly when used in the aortic or systemic atrioventricular (mitral) valve position, such that mechanical valves are usually used in these positions. A lower INR target of 1.5 to 2.0 for patients with an On-X bileaflet mechanical aortic valve and no additional thromboembolism risks can also be considered. Aspirin is recommended in patients regardless of whether additional anticoagulation is employed. There are no specific recommendations offered in regard to duration of aspirin therapy in this population. Tissue valves are generally considered to be more durable when placed on the right side for replacement of the pulmonary and tricuspid valves. For bioprosthetic pulmonary valves, which may be commonly used for pulmonary valve replacement in patients following tetralogy of Fallot (TOF) repair, for example, some centers use aspirin for 3 to 6 months postoperatively. Tricuspid valve replacement is less common, being used most commonly for Ebstein anomaly, followed by pulmonary atresia and TOF. For bioprosthetic tricuspid valves, it is reasonable to use warfarin to achieve an INR goal of 2.0 to 3.0, particularly if there is decreased ventricular function. Long-term aspirin is also a reasonable antithrombotic regimen for bioprosthetic tricuspid valves, often used in conjunction with warfarin.
Heparin-Induced Thrombocytopenia
HIT occurs in approximately 2% of adult patients and less than 1% of pediatric patients who are exposed to heparin. HIT is a hypercoagulable state resulting in the formation of immunoglobulin G antibodies produced against the complex of PF 4 on the surface of activated platelets and heparin, resulting in thrombocytopenia and significant platelet activation. Both clinical and laboratory tests are used to diagnose HIT. The clinical signs include thrombocytopenia (platelet drop of 50% within 24 hours) 5 to 8 days after the first heparin exposure (or sooner if heparin exposure has occurred in the past few weeks or months because HIT antibodies are transient). Two laboratory assays are available to detect HIT antibodies: platelet serotonin-release assay (SRA) and PF 4 -dependent enzyme immunoassays (EIAs). The SRA is the gold standard test because it has high sensitivity and specificity. The anti-PF 4 /heparin EIA is the laboratory test most commonly carried out, and it has the same high sensitivity as the SRA; however, a lower specificity results in a high false-positive rate (approximately 50% of patients clinically suspected to have HIT and a positive EIA actually have HIT type 2). In one study a positive EIA of more than 2.0 optical density (OD) units had approximately 90% predictivity for the presence of platelet-activating antibodies and a positive SRA. With an EIA of 1.4 OD units or higher, there was a 50% probability of the presence of platelet-activating antibodies.
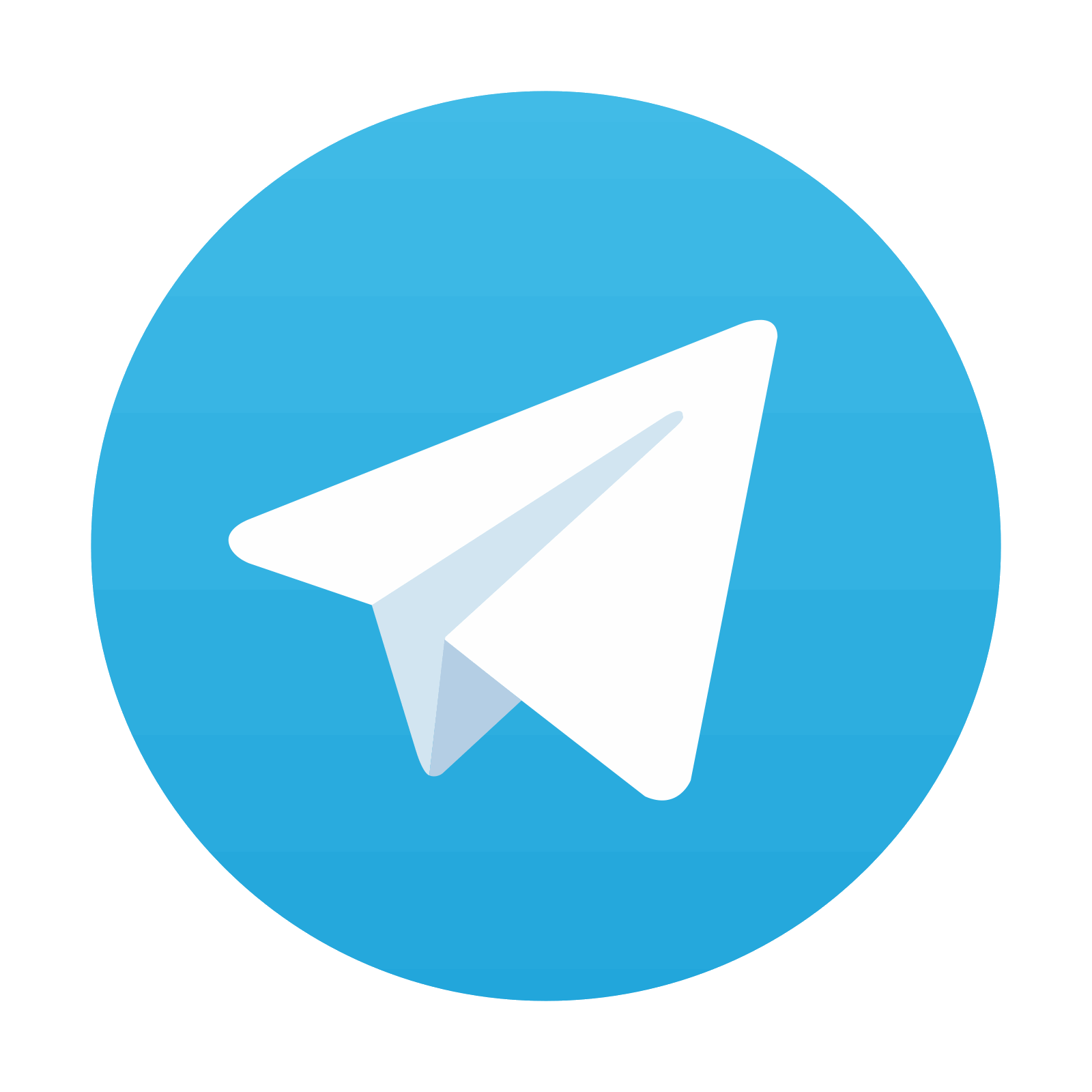
Stay updated, free articles. Join our Telegram channel
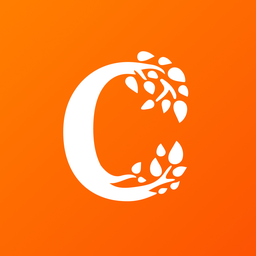
Full access? Get Clinical Tree
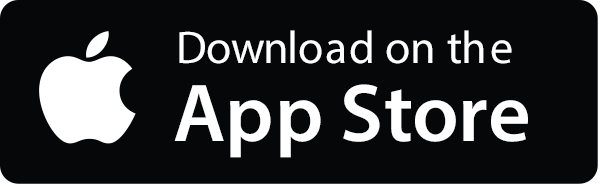
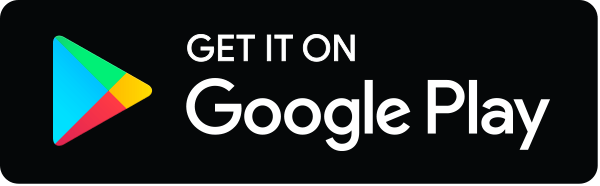