Antiarrhythmic drugs were developed with the expectation that they would extend and improve life for many patients with cardiovascular (CV) disease and those with a history of life-threatening arrhythmias. The usefulness of such drugs, however, has been limited by their ineffectiveness and/or toxicity. In mortality trials, benefit has not been clearly demonstrated, and worsened mortality has been observed with several drugs. A thorough clinical evaluation must therefore be conducted when deciding the mode of treatment or when deciding whether to treat at all.
The use of antiarrhythmic drugs has been dramatically altered by the findings of the Cardiac Arrhythmia Suppression Trial (CAST). This landmark study was designed to test the hypothesis that suppression of asymptomatic ventricular arrhythmias in patients with recent myocardial infarction (MI) would reduce mortality rate from cardiac arrest and arrhythmic sudden death. Prior to the CAST study, antiarrhythmic drugs were prescribed for these patients to suppress asymptomatic arrhythmias, a therapy aimed at reducing mortality. Based on the results of a feasibility and planning trial, the Cardiac Arrhythmia Pilot Study (CAPS), the CAST study evaluated encainide, flecainide, and moricizine. These drugs were chosen because they appeared to reasonably suppress symptomatic ventricular arrhythmias. In April 1989, the CAST study was interrupted by the Data Safety and Monitoring Committee, and encainide and flecainide were removed because they had been found to increase mortality rates twofold to threefold. The CAST II study continued to evaluate the remaining drug, moricizine; however, CAST II was also terminated prematurely, in August 1991, when it became apparent that moricizine was producing a similar trend toward harm and that there was no reasonable chance a beneficial effect on mortality could be detected. These results shocked the medical community but have influenced thinking in this and many other areas of medicine. Hine and colleagues reported a meta-analysis of CAST and similar studies with sodium channel–blocking antiarrhythmic drugs and found overall support for the conclusion of the CAST study, which has also led to recommendations by the Food and Drug Administration (FDA) for more restrictive labeling for all sodium channel–blocking antiarrhythmic drugs. In 1991, these drugs were given class labeling with indications for the treatment of documented ventricular arrhythmias that, in the judgment of the prescribing physician, are life threatening. Exceptions among sodium channel–blocking drugs are quinidine, propafenone, and flecainide, which have an additional indication for supraventricular arrhythmias.
With the loss of confidence in sodium channel blockers, attention turned to drugs that prolong cardiac refractoriness, such as amiodarone. Although these drugs are effective in controlling symptomatic arrhythmias, their effects on mortality rate have been unclear but seem neutral, except for an increase in noncardiac mortality in heart failure (HF) class III patients. Dofetilide, ibutilide, dronedarone, and the d-isomer of sotalol all prolong the action potential duration (APD) and were developed in the hope that they would have the efficacy of amiodarone but with a more favorable adverse event profile. However, the first of these drugs to be evaluated in a mortality trial, d-sotalol, was found to increase mortality rate after MI. Development of d-sotalol was halted, but the other two have been marketed with restrictions placed on their indications and clinical use. Clearly, antiarrhythmic drugs are some of the most complex drugs in clinical use today, and they require care in their selection and use.
Another development in the treatment of serious ventricular arrhythmias has been the automated internal cardioverter-defibrillator (ICD). The Antiarrhythmics Versus Implantable Defibrillators (AVID) trial found that devices are associated with a greater improvement in mortality rates. More recent evidence shows that ICDs reduce the rate of cardiac mortality and sudden death presumed to be ventricular tachyarrhythmic in case of sudden death in patients with HF, but ICDs have no effect on HF mortality.
In any case, patients with devices may continue to require treatment with antiarrhythmics, and interactions, both desirable and undesirable, between drugs and devices have been documented. In general, drugs that block sodium channels can increase pacing and defibrillation thresholds, and drugs that prolong refractoriness, such as potassium-blocking drugs, lower the defibrillation threshold.
Classification of Antiarrhythmic Drugs
Antiarrhythmic drugs are often classified according to their electrophysiologic effects. The scheme most often used was originally proposed by Vaughan Williams, who suggested it should be viewed as a class of drug actions that should be antiarrhythmic, not a classification of drugs. This is a subtle but important distinction that is made for the following reasons:
- •
Most antiarrhythmic drugs have multiple actions; hence their pharmacology is more complex than indicated by a simple drug-classification scheme.
- •
The actions of a given drug differ in the various kinds of cardiac tissues.
- •
Many antiarrhythmic agents have pharmacologically active metabolites, whose activity may be quite different in magnitude and in a class other than that of the parent compound.
- •
The relative amounts of these metabolites produced are genetically determined for several of these drugs, and they often vary extensively within the population.
Drugs having class I action possess “local anesthetic” or “membrane stabilizing” activity. Their predominant action is to block the fast, inward sodium channel. This produces a decrease in the maximum depolarization rate (V max ) of the action potential (phase 0) and slows intracardiac conduction. These agents have been further subclassified as belonging to either class IA, IB, or IC on the basis of their relative effects on specific aspects of intracardiac conduction and refractoriness. Drugs having class IA action include quinidine, procainamide, and disopyramide. These agents also produce measurable increases in ventricular refractoriness and prolongation of the QT interval. Lidocaine, mexiletine, and tocainide have actions belonging to class IB. Their potency for blocking sodium channels is only moderate, and in isolated tissues, they shorten the APD and refractoriness. At usual dosages, they generally exert little effect on PR, QRS, or QT intervals. Drugs with class IC actions are the more potent agents, flecainide and propafenone. Because these are potent sodium channel inhibitors, slowing conduction velocity but having little effect on repolarization, they increase the PR and QRS intervals at usual dosages. For these drugs, a prolonged QRS may be mistakenly interpreted as QT prolongation, but in fact these drugs with the exception of flecainide, cause little change in cardiac repolarization.
Class II action refers to β-adrenergic antagonism, possessed by agents such as propranolol, timolol, and metoprolol. Although these drugs are effective for treatment of supraventricular arrhythmias and tachyarrhythmias secondary to excessive sympathetic activity, they are not very effective in the treatment of severe arrhythmias, such as recurrent ventricular tachycardia (VT). With several proposed mechanisms of action, they are the only antiarrhythmic drugs found to be clearly effective in preventing sudden cardiac death in patients with prior MI.
Drugs whose predominant effect is to prolong the duration of the cardiac action potential and refractoriness have class III action: amiodarone, dronedarone, sotalol, bretylium, ibutilide, dofetilide, and N-acetylprocainamide (NAPA), the major metabolite of procainamide. It should be noted, however, that these drugs have mechanisms that overlap with other classes. Class IV action is calcium channel antagonism; antiarrhythmic drugs with this action include verapamil, bepridil, diltiazem, and nifedipine.
Because of the many limitations of the Vaughan Williams classification of antiarrhythmic drug mechanisms, another approach has been proposed, termed the Sicilian gambit . This classification system is based on the differential effects of antiarrhythmic drugs on 1) channels, 2) receptors, and 3) transmembrane pumps. The grouping is based primarily on the predominant action of drugs but also considers the other ancillary actions that may be clinically relevant. As shown in Figure 18-1 , because of the sequence of drugs listed, the symbols for these primary actions are generally aligned diagonally. For example, in this system quinidine is a sodium channel antagonist with potassium channel– and α-blocking activity. This provides a more complete and accurate description of the pharmacologic actions of the drugs than simply designating it as “class Ia.” When combined with an understanding of the electrophysiologic role of these actions, predictions about the effects likely to occur in vivo can be made more easily. In the case of quinidine, the three main actions produce conduction slowing, along with increased APD, and refractoriness, as well as vasodilation.

The Sicilian gambit also creates a framework in which newly discovered actions of drugs can be readily added. It emphasizes the multiple actions of drugs and the subtle differences and similarities that exist, and it is more complete. At present, the general understanding of the pharmacology of these drugs has progressed to the point that oversimplification can be misleading. The increased detail of this system reflects the current state of knowledge at a level necessary for optimal use of these drugs.
Because of the low level of efficacy of any one agent by itself, the treatment of acute or chronic ventricular arrhythmias frequently necessitates the use of multiple drugs, sequentially or in combination. For example, the astute clinician may produce increased sodium channel blockade and, hopefully, increase drug efficacy by using combinations of drugs with different kinetics of interaction with the sodium channel. Basic to these considerations is an understanding of the regulation of sodium channel function. Hodgkin and Huxley proposed that sodium channels exist in three distinct states: open, closed, and inactivated. According to the modulated receptor theory of cardiac sodium channel regulation proposed by Hille and by Hondeghem and Katzung, sodium channels in each of these states have differing affinities for drugs that block these channels.
This theory also provides a potential explanation for the phenomenon of “frequency dependence,” or “use dependence,” the increase in conduction block observed at an increasing rate of stimulation in response to sodium channel–blocking antiarrhythmic agents. Because an increase in the rate of stimulation increases the number of sodium channels in the open and inactivated states, antiarrhythmic agents that have greater affinity for activated (open) or inactivated channels, as opposed to rested channels, would have a greater opportunity to bind to the receptor and reduce conduction; therefore greater block will occur during tachycardia, leaving less drug action at normal heart rates. Also, antiarrhythmic drugs have different affinities for the different states of the sodium channel, and this is manifested as different rates for onset or recovery from block. Drugs that slowly associate with the receptor will cause block to accumulate over the first few cardiac cycles. Drugs that associate more rapidly, such as lidocaine, produce little additional block after the first beat in a train of stimuli. Likewise, drugs dissociate from the sodium channel at different rates, leading to differences in rates of recovery from block. The rate of onset of block of sodium channels has been proposed as a means to subclassify antiarrhythmic drugs. This is the electrophysiologic correlate of the subclassification of sodium channel blockers proposed by Harrison that was based on differences in clinical effects of the drugs.
This chapter reviews the clinical pharmacology and applications of the currently available antiarrhythmic drugs, excluding digoxin, β-receptor antagonists, and calcium channel blockers, which are addressed in other chapters. The drugs appear in the same order as listed in Figure 18-1 , an updated revision of the Sicilian gambit classification.
Drugs
Lidocaine
Clinical Applications
Introduced as a local anesthetic, lidocaine was first used as an antiarrhythmic agent in the 1950s for the treatment of arrhythmias arising during cardiac catheterization. Although lidocaine was historically used as a first-line antiarrhythmic agent for ventricular arrhythmias, this drug is now rarely recommended. It is considered a second choice behind amiodarone for the treatment of immediately life-threatening or symptomatic ventricular arrhythmias since publication of the ECC/AHA 2005 guidelines for cardiopulmonary resuscitation. Because extensive first-pass metabolism makes it unsatisfactory for oral use, congeners such as mexiletine were developed that would possess similar sodium channel–blocking actions and be active when taken orally.
Although lidocaine can be used for the acute suppression of ventricular arrhythmias, it has been shown to be ineffective for prophylaxis of arrhythmias in patients after MI. Because of lidocaine’s complex pharmacokinetics, a monitored environment is desirable to permit evaluation of patient response and detection of toxicity.
Lidocaine has little effect on atrial tissue in vitro, consistent with the clinical observation that it has no value in treating supraventricular tachyarrhythmias. Although lidocaine has been used to decrease the ventricular response during atrial fibrillation (AF) in patients whose atrioventricular (AV) conduction follows an accessory pathway, this is not an FDA-approved indication. In addition, some researchers have reported accelerated conduction and lack of efficacy in an unselected sample of AF patients.
Mechanism of Action
In concentrations similar to those attained during clinical use, lidocaine reduces V max and produces shortening or no change in APD and the effective refractory period of normal Purkinje fibers. This contrasts with quinidine and procainamide, which additionally block potassium channels and produce lengthening of APD. At usual concentrations, lidocaine has little effect on the electrophysiology of the normal conduction system, but in patients with abnormalities in this system, it has produced variable effects. Some studies have failed to detect significant changes in conduction, whereas others have found slowing of ventricular rate or potentiation of infranodal block in patients with conduction-system defects. Variability in dosage and pharmacokinetics may explain some of these discrepancies.
Clinical Pharmacology
Orally administered lidocaine is well absorbed, but it has poor bioavailability, because it undergoes extensive first-pass hepatic metabolism. Lidocaine clearance is well approximated by measurement of liver blood flow. The two desethyl metabolites, which are excreted by the kidneys, have less antiarrhythmic potency than the parent drug and may contribute to the production of central nervous system side effects that occur with lidocaine. Following intravenous (IV) administration, lidocaine’s biphasic disposition is well represented by a two-compartment pharmacokinetic model. Because antiarrhythmic activity is correlated with lidocaine’s concentration in the central compartment, and the half-life of distribution out of this compartment is rapid (~8 minutes), regimens that use a series of multiple loading doses and a maintenance infusion are able to achieve and then maintain a therapeutic concentration in plasma and myocardial tissue.
Regardless of the initial regimen used, during prolonged constant infusion, the lidocaine concentration eventually reaches a steady state dependent only on drug-infusion rate and lidocaine clearance. The time required to reach steady-state conditions is approximately 8 to 10 hours in normal individuals and up to 20 to 24 hours in some patients with HF or liver disease. This is longer than often anticipated because of the failure to recognize the relatively long elimination half-life: 1.5 to 2 hours in normal subjects and longer in patients with HF or hepatic disease.
Dosage and Administration
Lidocaine’s primary use is for acute and rapid suppression of highly symptomatic and life-threatening ventricular arrhythmias. Single IV boluses will achieve only transient therapeutic effects because the drug is rapidly distributed out of the plasma and myocardium; therefore, multiple loading doses should be used to rapidly achieve and sustain therapeutic plasma levels of lidocaine. Based on pharmacokinetic models validated in clinical studies, several regimens have been designed to maintain a relatively constant therapeutic level. For a stable patient, a total loading dose of lidocaine should be approximately 3 to 4 mg/kg body weight administered over 20 to 30 minutes. After injection of an initial dose of 1 mg/kg over 2 minutes, a series of three loading boluses can be administered slowly (approximately 50 mg each over 2 minutes) 8 to 10 minutes apart, while the patient is continuously observed for the development of side effects. Loading should be stopped if the transient, usually mild, central nervous system side effects persist, or if serious unwanted effects occur.
Another effective and well-tolerated loading regimen was suggested by Wyman and associates. For a 75-kg person, an initial bolus of 75 mg is recommended, followed by 50 mg every 5 minutes, repeated three times to a total dose of 225 mg. This regimen usually achieves and maintains plasma concentrations within usual therapeutic guidelines (1.5 to 5 µg/mL). A priming dose of 75 mg followed by a loading infusion of 150 mg over 18 minutes has also been used successfully. At the time of initiation of the loading regimen, a maintenance infusion should be started, designed to replace ongoing losses resulting from drug elimination. This may be calculated as the product of the desired plasma concentration (~3 µg/mL) and the expected clearance (see below). This calculation usually yields a dosage in the range of 20 to 60 µg/kg/min.
Even in normal individuals, wide variability occurs in peak plasma concentration; consequently, variability is seen in the calculated size of the central compartment for lidocaine. Therefore during loading, the patient’s electrocardiogram (ECG), blood pressure, and mental status should be monitored, and the process should be stopped at the first sign of lidocaine excess. When symptomatic arrhythmias persist in the presence of documented adequate dosage, defined by side effects or plasma concentration in excess of 5 to 7 µg/mL, another agent should be used.
If the maintenance infusion has reached steady state, but the concentration is below the level needed to prevent recurrence, and the arrhythmia reappears while side effects are absent, the appropriate actions are to 1) obtain a plasma sample for measurement of lidocaine concentration for future reference, 2) administer a small bolus of lidocaine (25 to 50 mg over 2 minutes), and 3) increase the maintenance infusion rate proportionally. The plasma concentration can be used to estimate clearance for calculation of the final maintenance infusion with the following formulas:
Maintenance dose = Clearance × Plasma concentration
Clearance = Infusion rate / Steady state plasma concentration
In effect, maintenance dose equals clearance multiplied by the desired plasma concentration, and clearance equals infusion rate divided by the plasma concentration measured at steady state. Little therapeutic effect is evident at lidocaine plasma concentrations below 1.5 µg/mL, whereas the risk of toxicity increases above 5 µg/mL. In some patients, however, concentrations in the range of 5 to 9 µg/mL may be required for arrhythmia suppression, which can safely be achieved with cautious drug administration.
Once steady state conditions have been achieved, simply terminating a lidocaine infusion will result in a gradual decline in plasma levels over the subsequent 8 to 10 hours as elimination occurs. Not only is there no reason to taper lidocaine infusions, but also it may be dangerous if oral antiarrhythmic therapy is initiated too early, because unpredictable additive effects may occur between lidocaine and newly started oral therapy. If a patient has reached steady state equilibrium, it is possible to estimate when the plasma lidocaine concentration will fall below the usual therapeutic levels. The plasma lidocaine concentration should be determined when the infusion is terminated, and the number of half-lives needed for that level to reach approximately 1.5 µg/mL can be estimated. The half-life of lidocaine for an individual patient can be estimated from the following equation, where V D is the final volume of distribution:
T 1 / 2 = Plasma concentration × V D × 0. 693 Infusion rate
The measured plasma concentration and the infusion rate are known components of the equation. V D is usually 1.1 L/kg, but it may be reduced by 50% or more in patients with HF.
Modification of Dosage in Disease States
Initial loading regimens require no adjustment in patients with renal or liver disease ; however, maintenance infusions must be decreased in liver disease and HF to compensate for decreased clearance. Because clearance alone is altered in liver disease with little change in the volume of distribution, the half-life of elimination is prolonged greatly, to as much as 5 hours, and steady state conditions may not be achieved until 20 to 25 hours after the institution of an IV infusion. Despite the fact that lidocaine metabolites are excreted by the kidneys, renal disease has not been reported to exert any significant effect on lidocaine dosing regimens, and dose adjustment may only be required in patients with severe renal insufficiency who are not receiving hemodialysis. With mechanical ventilation, cardiac output and hepatic blood flow are often decreased, so a decrease in lidocaine dosage may be required. Patients with congestive heart failure (CHF) achieve lidocaine levels that are almost double those in normal individuals administered the same dose. Because the central volume of distribution is generally halved in HF, loading doses should be reduced by 50%. Because clearance is also approximately halved, maintenance doses should be reduced proportionately from an infusion rate of 30 µg/kg/min for the typical patient to about half that. The time required to achieve steady state conditions following the initiation of a maintenance infusion is still 8 to 10 hours in many patients with HF because of concomitant changes in V D and clearance, resulting in a half-life similar to that seen in patients without HF.
In summary, general recommendations for initial lidocaine dosage selection should be adjusted for each patient based on clinical presentation and response and the results of plasma-level monitoring. Some patients with CHF may experience toxicity when given an infusion even as low as 0.5 mg/mL, so blood level monitoring is essential for proper dosage adjustment. In post-MI patients who receive lidocaine infusions for more than 24 hours, plasma lidocaine levels can increase, and the elimination phase half-life can increase up to 50%. This increase could be due in part to changes that occur in the protein binding of lidocaine during the first few days of therapy. Assays for plasma lidocaine measure the sum of both protein-bound and free lidocaine as total lidocaine and thus do not give a true picture of the amount of free drug available. An increase in plasma lidocaine occurring at this time often reflects an elevation in plasma levels of α-1–acid glycoprotein (AAG), to which it binds, which does not always indicate an increase in free, active drug. In this case, the lidocaine dosage should not be reduced to compensate for the higher total plasma concentration as long as the patient displays no adverse effects. Subsequent decreases in AAG concentrations will result in an apparent decrease in plasma lidocaine, which may reflect a drop in only the fraction bound to AAG.
Adverse Reactions
Central nervous system symptoms are the most frequent side effects of lidocaine administration. A rapid bolus can induce tinnitus or seizures. With more gradual attainment of excessive levels, drowsiness, dysarthria, confusion, hallucinations, and dysesthesia may occur. Excessive lidocaine can also cause coma, which should be a consideration in patients after cardiac arrest. Lidocaine can depress cardiac function, which decreases its clearance and produces an even greater increase in lidocaine concentrations. Advanced degrees of sinus node dysfunction have been reported in isolated instances. In patients with known conduction abnormalities below the AV node, lidocaine should be administered cautiously, if at all, unless a temporary pacemaker is readily available.
Drug Interactions
An additive or synergistic depression of myocardial function or conduction may occur when using lidocaine combined with other antiarrhythmic agents, especially during conversion from lidocaine to another antiarrhythmic agent. Lidocaine is metabolized in the liver into two active compounds, monoethylglycinexylidide (MEGX) and glycinexylidide (GX). The major metabolic pathway, sequential N-deethylation to MEGX and GX, is primarily mediated by CYP1A2 with a minor role for CYP3A4. A pharmacokinetic drug interaction between propranolol and lidocaine has been described experimentally and in humans in which β-adrenergic blockade caused decreases in cardiac output and liver blood flow with a resultant decreased lidocaine clearance. Lidocaine is considered a high hepatic extraction ratio drug, and cimetidine has been reported to reduce splanchnic, and hence hepatic, blood flow (see the Appendix at the end of this chapter); however, it appears that cimetidine has a greater effect on intrinsic hepatic metabolism of drugs as a result of CYP1A2 and CYP3A4 inhibition. Thus the effect of cimetidine on systemic clearance is less than the effect on oral clearance for a high extraction ratio drug such as lidocaine. Because lidocaine is administered parenterally, the magnitude of decrease in lidocaine clearance caused by cimetidine (12% to 25%) is unlikely to be of clinical significance. Nevertheless, patients who receive lidocaine should be monitored for the possibility of lidocaine toxicity, and both loading and maintenance dosages may require downward adjustment if cimetidine is added.
Mexiletine
Clinical Applications
Mexiletine is indicated for the treatment of documented ventricular arrhythmias, such as sustained ventricular tachycardia, that, in the judgment of the physician, are life-threatening. Initiation of therapy should be in the hospital with ECG monitoring. It also has been used successfully in the treatment of pain associated with diabetic neuropathy. Success rates vary between 6% and 60%, and more than half of the studies suggest limited efficacy (less than 20%). Mexiletine does not prolong the QT interval, it can therefore be useful for patients with a history of drug-induced torsades de pointes (TdP) or long QT syndrome when quinidine, sotalol, procainamide, or disopyramide are contraindicated. Although the rate of response to mexiletine is low when used alone, it has been combined successfully with quinidine, propranolol, or procainamide for ventricular arrhythmias. This mode of therapy takes advantage of the additive, and perhaps synergistic, antiarrhythmic response produced by the combination of these agents. Lower than usual dosages of both agents can be used, so that dosage-related adverse effects are reduced concomitantly. Mexiletine exerts minimal effects on both hemodynamics and myocardial contractility, even in patients with severe CHF.
Mechanism of Action
Mexiletine is an orally active lidocaine congener with class IB sodium channel–blocking activity and structural similarity to tocainide. It was originally developed as an anorexiant and anticonvulsant agent, and its antiarrhythmic properties were only later recognized. Mexiletine blocks fast sodium channels, decreasing V max and shortening the repolarization phase of ventricular myocardium.
Clinical Pharmacology
Mexiletine’s systemic bioavailability approximates 90%, with a large volume of distribution (5.5 to 9.5 L/kg) reflecting extensive tissue uptake. Approximately 1% of total body content of mexiletine is in the plasma compartment, with roughly 70% of this bound to serum proteins. Mexiletine has little first-pass metabolism but is eliminated primarily by hepatic metabolism, with only 10% to 15% being excreted unchanged in the urine. Its half-life of elimination is between 8 and 20 hours (9 and 12 hours for healthy subjects), with the time needed to reach steady state ranging between 1 and 3 days. Mexiletine undergoes extensive hepatic metabolism by CYP2D6 ; consequently, clearance is extremely variable (discussed later in this chapter).
Dosage and Administration
Mexiletine therapy should be initiated at a low dose and increased at 2- to 3-day intervals, until efficacy or intolerable side effects develop, such as tremor or other central nervous system symptoms. With normal renal function, the recommended initial oral mexiletine dosage is 200 mg every 8 hours. As with most drugs that have extensive liver metabolism, clearance will be widely variable within the general population. This is especially true for mexiletine, because CYP2D6, which is responsible for its metabolism, is absent in 7% to 9% of the white population. Also, it is necessary to consider dosage adjustment to compensate for the action of agents (discussed below) that induce or inhibit hepatic mexiletine metabolism.
Modification of Dosage in Disease States
Patients with renal failure who also are genetically deficient in hepatic CYP2D6 are likely to have extremely slow elimination for mexiletine. For this reason, all patients with renal failure should be given low initial doses. Elimination half-life and clearance may be prolonged by overt CHF and hepatic failure, and dosage reduction is required.
Adverse Reactions
The most frequently reported adverse events with mexiletine are gastrointestinal or neurologic in nature and include tremor, visual blurring, dizziness, dysphoria, nausea, dyspepsia, and, less frequently, dysphagia. Thrombocytopenia has been reported to occur infrequently with mexiletine therapy, and a positive antinuclear antibody test rarely occurs. Severe bradycardia and abnormal prolongation of sinus node recovery time have been reported in patients with the sick sinus syndrome, and at high concentrations, worsening of heart block has been reported. Oral mexiletine appears to have a reduced risk of causing worsened CHF compared with IV administration, which is not available in the United States.
Drug Interactions
Mexiletine’s hepatic metabolism can be increased by phenobarbital, phenytoin (Dilantin), or rifampicin, which reduce the half-life of mexiletine, possibly changing an effective dose to an ineffective one. Conversely, if treatment with an inducing agent is stopped, a previously effective dose may become toxic.
In one study, mexiletine decreased the clearance and increased plasma concentrations of theophylline. Quinidine inhibits the CYP2D6 enzyme primarily responsible for mexiletine’s metabolic clearance, and plasma concentration of mexiletine may increase in those individuals who express the enzyme—91% to 93% of whites.
Procainamide
Clinical Applications
Procainamide is indicated for the treatment of documented ventricular arrhythmias, such as sustained ventricular tachycardia, that, in the judgment of the physician, are life-threatening. Initiation of therapy should be in the hospital with ECG monitoring. Procainamide has been found to be useful in the acute management of patients with reentrant supraventricular tachycardia, AF, and the flutter associated with Wolff-Parkinson-White syndrome.
Procainamide has also been used intravenously to suppress ventricular arrhythmias that occur immediately following MI or to convert sustained VT. One randomized study found procainimide (10 mg/kg) to be superior to lidocaine (1.5 mg/kg) for termination of hemodynamically stable VT. However, because it takes approximately 20 minutes to administer a loading dose of procainamide safely, its use is limited to situations when adequate time is available. An advantage over lidocaine is the potential for conversion to oral therapy using the same agent.
The active metabolite of procainamide, N -acetylprocainamide (acecainide [NAPA]), produces class III antiarrhythmic activity in some patients, although not always in those who respond to procainamide. This is most likely due to the very different electrophysiologic actions of procainamide and NAPA. NAPA was investigated as an antiarrhythmic drug and was shown to be effective in the treatment of some types of ventricular arrhythmias, but its use was limited by a narrow therapeutic index, and development was therefore halted.
Mechanism of Action
As with other agents that demonstrate class I activity, procainamide slows conduction and decreases automaticity and excitability of atrial and ventricular myocardium and Purkinje fibers. Because of its effect on potassium channels, it also prolongs APD and refractoriness. Compared with quinidine, procainamide has very little vagolytic activity and does not prolong the QT interval to as great an extent. NAPA has predominantly class III antiarrhythmic activity; it prolongs APD and refractoriness in both atrial and ventricular myocardium and prolongs the QT interval. It has little or no effect on V max , in either Purkinje fibers or ventricular cells, and it does not alter His-Purkinje conduction velocity because of its very low potency as a sodium channel antagonist.
Clinical Pharmacology
Procainamide is rapidly absorbed and 100% orally bioavailable. About 15% of procainamide is bound to serum proteins, and its short elimination half-life of 2 to 4 hours in patients with normal renal function necessitates dosing every 3 to 6 hours. Dosing every 6, 8, or 12 hours is possible with sustained-release preparations. The varied formulations and their very different dosing requirements often create confusion and can lead to dangerous mistakes in dosing.
Slightly more than half of the general population are phenotypic rapid acetylators of procainamide and quickly convert it to NAPA, a metabolite with very pure class III antiarrhythmic action. As would be expected, however, the response to one agent does not predict response to the other. When each is given as the sole agent, the plasma concentration that is usually effective is 4 to 8 µg/mL for procainamide and 7 to 15 µg/mL for NAPA. During oral procainamide therapy, both agents are present in variable amounts, and there is no way to readily determine the contribution of NAPA to arrhythmia suppression under these conditions. Consequently, the utility of measuring plasma levels of procainamide during chronic therapy is limited because of this variable hepatic conversion to NAPA; however, monitoring plasma concentrations for determination of compliance or prevention of toxicity is both feasible and recommended (see below).
Dosage and Administration
Procainamide is available for either IV or oral use. For patients with normal renal and cardiac function, the initial recommended oral maintenance dose is 50 mg/kg/day. Frequent administration is required for oral procainamide, which is inconvenient and makes compliance difficult. Sustained-release forms of procainamide are available that permit dosing every 6, 8, or 12 hours, depending upon the formulation. During long-term therapy, levels of NAPA may accumulate to effective or toxic levels in some individuals, resulting in achievement of maximum pharmacologic effect long after procainamide has reached steady state. Therefore, the elimination half-life of 2 to 4 hours for procainamide may be misleading as a predictor of time to occurrence of stable pharmacologic action. Thus dosage should be initiated at conservative levels, and the patient should be carefully monitored until both procainamide and its metabolite have reached a steady state. Because the electrophysiologic effects of procainamide and NAPA are quite different, monitoring patients receiving procainamide should at some point include measurement of plasma concentrations of both agents to determine their relative concentrations. Patients who are rapid acetylators or who have impaired renal function usually have plasma concentrations of NAPA that are higher than procainamide at steady state. These individuals should be monitored for excessive accumulation of NAPA during dose titration to maintain plasma levels of NAPA below 20 µg/mL. The practice of using the sum of the plasma concentration of procainamide and NAPA is without basis and is not recommended.
When administered IV, procainamide can be given as a constant 25-minute loading infusion of 275 µg/min/kg or by a series of doses (100 mg delivered over 3 minutes) given every 5 minutes, up to a total dose of 1 g. If the loading infusion is well tolerated, with no hypotension and less than 25% QRS or QT widening, a maintenance IV infusion of 20 to 60 µg/kg/min can then be given. Larger and more rapid loading infusions of 1 g over 15 to 20 minutes have been given in the electrophysiology laboratory to prevent induction of VT by programmed ventricular stimulation. A second loading infusion of 0.5 to 1 g has been given in some instances when an initial loading infusion was well tolerated but ineffective, but these large dosages are accompanied by a higher incidence of hypotension and conduction disturbance and often result in attainment of unacceptably high plasma concentration.
Modification of Dosage in Disease States
With renal dysfunction or a low cardiac output, both procainamide and NAPA in the usual doses may accumulate to potentially toxic levels, and the dose should be reduced. Increased plasma levels of procainamide and/or NAPA may occur with CHF because of decreased urinary excretion and hydrolysis of procainamide. However, two studies of procainamide pharmacokinetics following a single IV bolus revealed no difference in volume of distribution, clearance, elimination half-life, unbound drug fraction, and peak procainamide concentrations between patients with CHF and normal individuals. Although IV procainamide does depress myocardial contractility and also lowers blood pressure, worsening of HF is uncommon during oral therapy, when the usual dosages and plasma concentrations are maintained.
Adverse Reactions
Side effects associated with long-term procainamide therapy limit its usefulness. Up to 40% of patients discontinue therapy in the first 6 months because of adverse reactions. The potential exists for arrhythmia aggravation, including the development of TdP with procainamide or, more often, due to NAPA. Therefore, as with all agents that possess class IA activity, procainamide should not be used in patients with long QT syndrome, a history of TdP, or hypokalemia. To reduce the occurrence of proarrhythmia, potassium levels should be maintained above 4 mEq/L when taking procainamide. Heart block and sinus node dysfunction can occur in patients with preexisting conduction system abnormalities.
Between 15% and 20% of patients who receive long-term oral procainamide therapy develop a lupuslike syndrome that is often difficult to recognize but regresses with discontinuation of treatment. If necessary, corticosteroid treatment may be used to treat unresolved symptoms after drug withdrawal. The syndrome usually begins insidiously as mild arthralgia but progresses to frank arthritis, fever, malar erythematous rash, and pleural and/or pericardial effusions, with serum antibodies against nucleoprotein (histone) appearing as antinuclear antibodies with a smooth or diffuse pattern. These symptoms abate if procainamide is discontinued and generally resolve at a rate proportional to their duration. Approximately 80% of patients who receive prolonged therapy with the drug demonstrate an increased titer of antinuclear antibodies, early evidence of lupus, usually within 1 to 12 months of therapy initiation, but only 15% to 20% develop symptoms of the lupus syndrome. Therefore, it is unnecessary to discontinue therapy solely because of the positive antinuclear antibody titer. Slow acetylation status may indicate a higher risk for lupus symptoms or an increased rate of development of antinuclear antibodies, especially in patients with renal impairment. The patient should be fully informed of the symptoms, which should be reported, so therapy can be discontinued at the earliest signs or symptoms of the syndrome. Continuing procainamide after the development of the early symptoms of the lupus syndrome is dangerous because of the possibility of pleural effusion and potentially lethal pericardial tamponade.
Procainamide therapy has also been associated with the development of agranulocytosis. Although more research is needed, it has been suggested that the sustained-release form of the drug may be especially capable of inducing this toxicity. The manufacturer recommends that a white blood count be obtained every week for the first 3 months and periodically thereafter.
Drug Interactions
Unlike quinidine, procainamide does not cause an increase in digoxin levels. Its clearance is reduced between 30% and 50% by cimetidine, which blocks the renal tubular secretion of procainamide. A similar competition has been found between procainamide and its predominant metabolite, NAPA. Ranitidine affects procainamide pharmacokinetics by reducing both its renal clearance and its absorption, the former by 14% to 23% and the latter by 10% to 24%, depending on the dose.
Disopyramide
Clinical Applications
Disopyramide is effective against a broad range of supraventricular and ventricular arrhythmias, but it is only approved by the FDA for treatment of documented ventricular arrhythmias, such as sustained ventricular tachycardia, that, in the judgment of the physician, are life-threatening. Initiation of therapy should be in the hospital with ECG monitoring. Its antiarrhythmic profile is similar to that of quinidine and procainamide; although newer than these, disopyramide is still one of the older antiarrhythmic agents, having been in use in the United States since 1977. Its negative inotropic and anticholinergic actions occur frequently and limit its usefulness.
Mechanisms of Action
Disopyramide’s class IA antiarrhythmic effects are predominantly those associated with sodium and potassium channel blockade. Its effects are similar to those of quinidine and procainamide on automaticity, conduction, and refractoriness in atrial and ventricular tissue.
Clinical Pharmacology
Disopyramide’s oral bioavailability is 80% to 90%. Its half-life of elimination, usually 6 to 8 hours, is lengthened to as long as 15 hours in cardiac patients. About half of the compound is eliminated by the kidneys unchanged, and the remainder is eliminated as an active metabolite resulting from hepatic N -dealkylation. Protein binding of disopyramide is complex, with between 20% and 50% of disopyramide being bound to plasma proteins. For most drugs, the percentage bound to plasma protein is a constant over the usual range of therapeutic concentrations. The saturation of disopyramide-binding sites on plasma proteins at usual doses means increases in levels of free drug in plasma are disproportionate compared with the magnitude of dosage increment.
Dosage and Administration
Loading doses are not recommended with disopyramide. The usual effective dosage for disopyramide is 100 to 200 mg three to four times daily, up to a maximum dose of 800 mg/day. Dosages up to 1600 mg/day have been required for some refractory arrhythmias. Therapy should be very carefully titrated, beginning with low doses and allowing ample time for achievement of steady state equilibrium.
Although rapid fluctuations in plasma concentration are undesirable, they are difficult to avoid because of disopyramide’s saturable protein binding. The controlled-release form of disopyramide may be useful in reducing adverse effects by decreasing fluctuations in the concentration of free disopyramide in plasma. Because of saturable protein binding, the generally accepted therapeutic range for total disopyramide in plasma of 2 to 5 µg/mL should not be relied on with confidence. Although monitoring the plasma concentrations of free disopyramide has been recommended, the range of concentrations associated with arrhythmia suppression has not been clearly delineated and overlaps with that associated with adverse effects.
Modification of Dosage in Disease States
Patient response to disopyramide should be monitored especially closely after acute MI, when both the absorption and elimination of disopyramide are decreased. In fact, in view of disopyramide’s negative inotropic actions and changes in levels of binding proteins in plasma following MI, other antiarrhythmic agents should be considered first.
Disopyramide is contraindicated in patients with uncompensated HF because it can worsen such failure. The initial dosage of disopyramide should be reduced to 50 to 100 mg every 12 hours in patients with renal insufficiency or decreased hepatic function.
Adverse Reactions
The predominant side effects of disopyramide include new or worsened CHF and symptoms resulting from dose-related anticholinergic actions, including urinary retention, constipation, dry mouth, and esophageal reflux. Because of this anticholinergic action, patients with obstructive uropathy or glaucoma should not take this agent. For some patients, the anticholinergic side effects can be prevented or alleviated by concomitant use of cholinesterase inhibitors such as physostigmine and neostigmine without reduction in antiarrhythmic efficacy. As with all agents that prolong repolarization, disopyramide should not be used in patients with long QT syndrome, hypokalemia, or a history of TdP because of the potential for arrhythmia aggravation. Direct actions of disopyramide on the sinus node can lead to excessive bradycardia in patients with sinus node dysfunction, which may contribute to development of TdP in patients with hypokalemia.
Drug Interactions
Disopyramide does not increase digoxin levels, and the effects of warfarin are not potentiated by disopyramide. Phenytoin, rifampicin, and phenobarbital induce CYP3A4, thus enhancing hepatic metabolism of disopyramide, which leads to an increase in its elimination and to the potential loss of antiarrhythmic effect. Significant depression of myocardial contractility may result from the combined administration of disopyramide with β-adrenergic or calcium channel antagonists; this combination should be avoided in patients with impairment of ventricular function.
Quinidine
Clinical Applications
Quinidine is an old drug that, in the past, has been used successfully for a variety of supraventricular and ventricular arrhythmias, including conversion of AF or atrial flutter, supraventricular tachycardia, VT, and ventricular fibrillation. On the other hand, a grouped analysis of six small placebo-controlled trials in patients with AF showed a statistically significant increase in mortality rate for the patients treated with quinidine, and another meta-analysis also showed a threefold increased mortality rate with quinidine. Because of the similar negative effects on mortality seen in CAST and CAST II, quinidine’s risk/benefit ratio must be carefully considered in each patient. The FDA recommends it only in refractory patients for conversion of symptomatic AF or flutter, prevention of recurrences of AF, and the treatment of documented ventricular arrhythmias, such as sustained ventricular tachycardia, that, in the judgment of the physician, are life-threatening.
Mechanism of Action
Quinidine has multiple actions, but the action thought by many to be primarily responsible for its efficacy is block of the rapid inward sodium channel. This results in a decrease in V max of the action potential upstroke and slowed conduction that is more marked in the His-Purkinje system than in the atria. Quinidine’s effects on sodium channels are greatest at increased heart rate and less negative membrane potential—that is, they are pH, rate, and voltage dependent. Dose-related changes in the ECG are manifested as increases in PR, QRS, and QTc intervals, which reflect quinidine’s multiple actions.
Clinical Pharmacology
Quinidine’s effective dosage varies among individuals because of several factors. Although quinidine sulfate is usually administered every 6 hours, wide interindividual differences are seen in its elimination half-life, which varies from 3 to 19 hours. Plasma protein binding also varies widely, ranging from 50% to 95%. Oral bioavailability is approximately 70%, and clearance after oral administration ranges from 200 to 400 mL/min. Quinidine is inactivated or eliminated by both hepatic metabolism (50% to 90%) and renal elimination (10% to 30%). Several potentially active metabolites are formed in amounts that vary among individuals, but for most, their clinical role has not been determined. One of quinidine’s metabolites, 3-hydroxyquinidine, has been shown to possess antiarrhythmic activity when given to humans. Experimental data indicate some contribution by metabolites of quinidine to its antiarrhythmic action.
Dosage and Administration
Quinidine therapy (as the sulfate) is usually initiated with an oral dosage of 200 mg every 6 hours, and the dosage is titrated every 3 or more days. Elderly patients often require lower dosages of quinidine because of both reduced clearance and volume of distribution.
Quinidine is available commercially in at least two different forms: quinidine sulfate and quinidine gluconate. Because the quinidine content varies between these at 83% and 62%, respectively, the need for dose adjustment should be considered if one form is substituted for another. The usually effective dosage of quinidine sulfate ranges from 800 to 2400 mg/day, with the maximum recommended single dose being 600 mg. Because the half-life varies from 3 to 19 hours, a delay of 4 days between dosage increases is recommended to prevent unexpected drug accumulation. The range of therapeutic plasma concentrations measured using assays that differentiate quinidine from its metabolites is 0.7 to 5.5 µg/mL. Rapid escalation in quinidine dosage has been used to convert AF, but this therapy is no longer recommended because of unnecessary toxicity.
IV therapy with quinidine is usually avoided if alternatives are feasible. Vasodilation and hypotension result from quinidine-induced α-adrenergic blockade. If quinidine is administered intravenously as quinidine gluconate, the patient should be carefully monitored, and the infusion rate should be no more rapid than 16 mg/min. The infusion should be discontinued if hypotension is observed, or if the QRS is prolonged by more than 30%.
Modification of Dosage in Disease States
No adjustment in initial dosage is usually needed for patients with renal or hepatic disease, although because of decreased protein binding in patients with hepatic failure, lower than usual total plasma concentration can produce toxicity. Slower dose titration is advisable to permit attainment of steady state and complete accumulation of active metabolites; however, because the usual range of effective dosages is wide, dosage for these patients is not markedly different. Patients with rapid quinidine elimination may require higher dosages, up to 600 mg every 6 hours. This is often due to induction of hepatic metabolism caused by other drugs.
Patients with congenital long QT syndrome, hypokalemia, or a history of TdP should not be given quinidine because of their increased risk for this form of proarrhythmic event. For patients with CHF, more serious problems associated with use of quinidine are proarrhythmia and digitalis toxicity, either with digitoxin or digoxin. Prudent use of quinidine in individuals taking digitalis requires 1) that titration begin at a reduced dosage; 2) that dosage of any cardiac glycoside being administered concomitantly be reduced; and 3) that plasma electrolyte levels, especially potassium, be maintained above 4 mEq/L.
Although quinidine does possess some direct negative inotropic effects, these are usually counteracted by its vasodilatory effect; therefore, oral quinidine is well tolerated hemodynamically when given at dosages that produce usual plasma concentrations, even in patients with reduced ventricular function. In a study of more than 650 patients, 35% of whom had CHF, quinidine therapy resulted in no induction or worsening of CHF. On the other hand, a significant problem for patients with CHF who receive quinidine therapy is proarrhythmia, with quinidine-induced TdP being potentiated in the setting of bradycardia and low serum levels of magnesium or potassium.
Adverse Reactions
Marked prolongation of the QT interval has been seen in some patients receiving low or usual dosages of quinidine, and the risk of TdP is markedly increased. This arrhythmia may be responsible for all cases of quinidine-induced death and quinidine-induced syncope, which occur in as many as 5% to 10% of patients within the first days of quinidine treatment. TdP usually occurs in patients with low concentrations of quinidine, hypokalemia, hypomagnesemia, poor ventricular function, and bradycardia, and females have an increased susceptibility. In a study by Drici and colleagues, dihydrotestosterone reduced the sensitivity to the effects of quinidine on the QT interval in animals. Additional studies showed women to be more sensitive to the effects of quinidine on the QT interval, providing evidence that sex hormones have direct effects on cardiac tissue that may be responsible for the differences in baseline QT interval and incidence of TdP in men and women.
The first steps for the treatment of patients who develop TdP are to stop medications known to prolong the QT interval and to correct electrolyte imbalances. Observational studies support the use of magnesium sulfate (2 g IV bolus with 2 to 4 g repeated once, if necessary) to treat TdP. The efficacy of magnesium is supported by the findings of a prospective controlled trial of two 5-g doses of magnesium that showed it reduced the incidence of TdP and improved the efficacy of ibutilide in 476 patients with AF or flutter. One case series showed that isoproterenol or ventricular pacing can be effective in terminating TdP associated with bradycardia and drug-induced QT prolongation. It is essential to clinically distinguish TdP from polymorphic VT in the setting of a normal QT interval, because in this setting, magnesium is likely to be ineffective. Because quinidine acts via α-adrenergic blockade to induce vasodilation, hypotension may occur, especially in patients concomitantly receiving nitrates or other vasodilators. Other adverse effects include a high incidence of diarrhea and vomiting, tinnitus at high plasma levels, thrombocytopenia, and conduction block in patients with existing conduction-system disease. In patients treated with quinidine for atrial flutter without prior AV nodal blockade by digitalis, there have been reports of sudden increases in AV conduction and rapid ventricular rates. This is the result of a slight reduction of the flutter rate and enhanced AV nodal conduction because of the anticholinergic effects of quinidine. This allows one-to-one conduction through the AV node, often at 200 to 250 beats/min. This may be of particular concern for patients receiving other drugs that prolong conduction time through the AV node, such as β-adrenergic agonists.
Drug Interactions
Quinidine metabolism is inhibited by cimetidine and induced by phenytoin, phenobarbital, and rifampicin, with the latter agents leading to reduced, often subtherapeutic quinidine concentrations. Clinical digoxin toxicity has been described in 20% to 40% of patients receiving quinidine and digoxin concurrently. The magnitude of this interaction is dependent on quinidine dosage, and in some patients, it may not appear until the dosage is increased to higher levels. The rise in digoxin levels appears with the first dose of quinidine, therefore it is suggested that digoxin dosage be halved when quinidine therapy is initiated. A similar interaction has been reported for quinidine and digitoxin.
Although quinidine is a substrate of the hepatic cytochrome P450 (CYP) 3A4 isozyme, it is a potent inhibitor of CYP2D6. Thus, it may interfere with the biotransformation and actions of pharmacologic agents dependent on this cytochrome for their metabolism, which include propafenone, mexiletine, flecainide, metoprolol, timolol, sparteine, and bufuralol. Quinidine worsens neuromuscular blockade in patients with myasthenia gravis and may prolong the effects of succinylcholine.
Propafenone
Clinical Applications
Propafenone was developed in Germany, where it has been marketed since 1977. Propafenone is used primarily to treat atrial arrhythmias, paroxysmal supraventricular tachycardia, and ventricular arrhythmias in patients with no or minimal heart disease and preserved ventricular function.
Clinical Pharmacology
Propafenone has a marked structural similarity to propranolol, and studies have shown that propafenone can accumulate during continued administration to levels capable of producing clinically significant β-adrenergic inhibition.
Like mexiletine and flecainide, propafenone is eliminated by a metabolic pathway that has a polymorphic pattern of inheritance. Patients deficient in CYP2D6 activity have very slow elimination of propafenone and fail to form measurable quantities of the potentially active metabolite, 5-hydroxypropafenone. The accumulation of high concentrations of propafenone leads to significant β-receptor antagonism at both low and high doses in poor metabolizers but only at high dosages in extensive metabolizers of propafenone. Although metabolic phenotype does not seem to dramatically influence the antiarrhythmic response to propafenone in many patients, it clearly influences the degree of β-blockade that occurs during therapy.
Dosage and Administration
Effective dosages range from 300 to 900 mg/day in two to four divided doses. To prevent unexpected accumulation of pharmacologic action, propafenone dosage should not be changed more frequently than every 3 days; elimination of the parent drug is slow in poor metabolizers, and accumulation of the metabolites is slow in extensive metabolizers.
Patients with reduced ventricular function, especially those receiving propafenone, should be carefully monitored for deterioration in ventricular function, which may result from β-adrenergic receptor antagonism and/or the direct negative inotropic effect.
Modification of Dosage in Disease States
A reduction of approximately 20% to 30% in the normal oral dosage for immediate-release tablets is recommended for patients with hepatic dysfunction; no reduction is recommended for patients with renal insufficiency. Severe liver dysfunction increases propafenone bioavailability to approximately 70% compared with 3% to 40% for normal hepatic function. Although specific guidelines are not available, the manufacturer recommends consideration of dosage reduction for the extended-release capsules (Rythmol SR) in patients with hepatic impairment.
Drug Interactions
It is very likely that drug interactions will occur between propafenone and other agents that utilize or inhibit CYP2D6 for their metabolism. Such an interaction has been documented already between propafenone and metoprolol and should be expected with timolol, many antidepressants, many neuroleptics, and perhaps other agents. Quinidine, which inhibits this cytochrome, inhibits the formation of 5-hydroxypropafenone in extensive metabolizers; however, the clinical consequence of such inhibition is unknown and difficult to predict. Greater β-blockade occurs after combining quinidine with propafenone therapy because of the resulting higher propafenone concentrations and its actions to block β receptors.
Flecainide
Clinical Applications
As a result of the CAST study, flecainide is not considered a first-line agent in patients with structural heart disease because of its propensity for fatal proarrhythmic effects.
Like propafenone, flecainide is used primarily to treat atrial arrhythmias, paroxysmal supraventricular tachycardia, and ventricular arrhythmias in patients with no or minimal heart disease and preserved ventricular function.
Mechanism of Action
Flecainide has sodium channel–blocking activity and is considered to have class IC actions. It has also been found to block the delayed rectifier potassium channel in feline ventricular myocytes, and this action may be clinically relevant.
Flecainide slows intraventricular conduction velocity more than it prolongs effective refractory periods. It prolongs A-H and H-V intervals and measurably increases PR and QRS intervals on the surface ECG at therapeutic doses. The QTc interval is slightly increased, primarily as a result of prolongation of the QRS, but its ability to block the delayed rectifier potassium channel may contribute to QT changes.
Clinical Pharmacology
The systemic bioavailability of oral flecainide is 90% to 95%, and most of the flecainide is metabolized in the liver into compounds that are not pharmacologically active at the concentrations usually found in plasma. Like many other antiarrhythmic agents, flecainide is metabolized by CYP2D6. Because flecainide is also eliminated by the kidneys to a considerable extent, the enzyme deficiency has little effect on its pharmacokinetics; however, if those patients without the enzyme develop renal insufficiency, or when patients with renal failure are given a drug that blocks flecainide’s metabolism, extremely high plasma concentrations are likely to occur. Flecainide has a very slow elimination, with a half-life that ranges from 7 to 23 hours in normal individuals and tends to be even longer, from 14 to 26 hours, in patients with cardiac disease, even in the absence of HF.
Dosage and Administration
After an initial dose of 100 mg every 12 hours, maximum dosage of flecainide for ventricular arrhythmias is 150 mg every 12 hours in patients without cardiac or renal failure. The recommended dose for patients with supraventricular tachyarrhythmias is 50 or 100 mg every 12 hours as a starting dose. The range of therapeutic plasma concentrations of flecainide is reported to be between 200 and 1000 ng/mL. However, adverse effects may occur in some patients at concentrations within this range, although other patients may tolerate concentrations well above it. To reduce the incidence of adverse effects, flecainide therapy should start with a low dose that is maintained until steady state has been reached (at least 4 days) and altered relative to clinical response.
Modification of Dosage in Disease States
With cardiac failure, the usual initial dose is 50 to 100 mg every 12 hours. Because 7% to 9% of white patients with renal failure will not have the CYP2D6 enzyme, and because flecainide is usually eliminated by both metabolism and renal excretion, all patients with renal failure should be given very low dosages, titrated very slowly and carefully. Plasma concentration monitoring is essential in patients with renal disease or cardiac or hepatic dysfunction. Any significant reduction in ejection fraction should be expected to lengthen elimination half-life and hence the time needed to attain steady state equilibrium, and reductions in clearance may occur in renal or hepatic dysfunction that lead to higher plasma concentrations at steady state.
Adverse Reactions
Flecainide has the potential to induce proarrhythmic events, even when prescribed as recommended. This is especially true in patients with severe heart disease, and when flecainide is given in higher dosages. Because of its negative inotropic effects at dosages necessary to suppress arrhythmias, flecainide produces a measurable decrease in left ventricular (LV) function in most patients. Because of the increased mortality rate seen in the CAST study, flecainide is not considered a first-line agent because of its propensity for fatal proarrhythmic effects. Additionally, its negative inotropic actions restrict its use to patients with moderately well-preserved ventricular function.
Other side effects of flecainide include depression of sinus node activity in patients with preexisting sinus node dysfunction and prolongation of QRS and PR intervals on the surface ECG. In addition, flecainide increases pacing thresholds by as much as 200% and should therefore be used with caution in patients dependent on pacemakers. It also increases the threshold for electrical defibrillation, so patients with implanted devices should be evaluated carefully.
Drug Interactions
Cimetidine reduces flecainide clearance and prolongs its elimination half-life. Studies in normal volunteers have shown an increase in the plasma concentrations of digoxin and propranolol when flecainide is coadministered. Not unexpectedly, propranolol and flecainide have been found to have additive negative inotropic effects. An interaction with amiodarone has been described that results in elevation of plasma flecainide concentration and necessitates reduction of flecainide dosage.
Sotalol
Clinical Applications
Sotalol is a unique β-blocker that shows class II and III antiarrhythmic effects, but as a nonselective β-blocker, it lacks intrinsic sympathomimetic and membrane-stabilizing activity. Like amiodarone, sotalol prolongs the APD and increases refractoriness. Sotalol is used in the treatment of atrial arrhythmias or life-threatening ventricular arrhythmias, including sustained VT. However, it should not be used for minor arrhythmias, because it is known to be proarrhythmic with an increased risk for TdP. Although sotalol is effective for the maintenance of sinus rhythm in patients with AF, amiodarone was shown to be superior for maintaining sinus rhythm in this population, as data from the Solatol Amiodarone Atrial Fibrillation Efficacy Trial (SAFE-T), the Clinical Trial of Atrial Fibrillation (CTAF), and the Atrial Fibrillation Follow-up Investigation of Rhythm Management (AFFIRM) indicate.
Nonetheless, it has not yet been determined whether the survival rate is improved with sotalol use versus use of an implanted defibrillator alone. Sotalol was originally approved by the FDA under the brand name Betapace for the treatment of life-threatening ventricular arrhythmias in October 1992. The d-isomer of sotalol—with virtually no β-blocking activity, and considered to have only class III antiarrhythmic effects—was studied as an antiarrhythmic in high-risk patients after MI in the Survival With Oral d-Sotalol (SWORD) trial, but the study was terminated prematurely because of a higher mortality rate in the d-sotalol group compared with the placebo group. This finding was presumably due to increased arrhythmic deaths. The present commercial product, Betapace AF, is intended for use in AF, with the caveat that sotalol is not effective for conversion of AF to sinus rhythm, but it may be used to prevent AF. Although both commercial presentations contain sotalol, Betapace should not be substituted for Betapace AF because of significant differences in the labeling sections on indications, dosing, administration, and safety profile. The injectable form of sotalol was approved by the FDA in July 2009.
Although sotalol can also be used to treat paroxysmal supraventricular tachycardia, the higher risk for proarrhythmia makes AV nodal blocking agents—such as adenosine, calcium channel blockers, and β-blockers—more desirable agents, with the exception of patients with preexcited atrial arrhythmias, in whom such AV blockers are contraindicated.
Mechanism of Action
In addition to its β-blocking effects, sotalol markedly prolongs refractoriness in atrial and ventricular tissues, a class III antiarrhythmic action. These actions slow heart rate, decrease AV nodal conduction, and increase refractoriness of atrial, ventricular, AV nodal, and AV accessory pathways in both the anterograde and retrograde directions. When given in dosages between 160 and 640 mg/day, increases of 40 to 100 ms are seen in the QT interval, and 10 to 40 ms increases are observed in the QTc.
Clinical Pharmacology
Oral bioavailability of sotalol is greater than 90%, and peak concentrations are seen 2.5 to 4 hours after a dose. It is not bound to plasma proteins and is eliminated by the kidneys unchanged, with an elimination half-life of approximately 12 hours. Because of the relatively long half-life and the twice-daily dosing regimen, it is recommended that testing for efficacy be conducted near the end of the dosing interval at steady state. Age, per se, does not influence the pharmacokinetics of sotalol other than those effects that are due to the natural decline in renal function that occurs with age.
Dosage and Administration
The recommended initial oral dose of sotalol is 80 mg every 12 hours. In patients with relatively normal renal function, steady state is reached in 2 to 3 days. If evaluation at this dosage indicates a lack of desired response without evidence of excessive effects on repolarization (QT >500 ms), the dosage may be increased to 160 mg twice daily and, if necessary, to 240 mg twice daily. Some patients with life-threatening arrhythmias have required dosages of 640 mg/day. Accelerated titration regimens have been used with close monitoring without any apparent increase in adverse events.
It is recommended that before starting therapy or increasing the dose of sotalol, the QT interval, heart rate, and creatinine clearance (CLCR) be evaluated. IV sotalol is contraindicated if the baseline QT is greater than 450 ms, heart rate is less than 50 beats/min, or if CLCR is less than 40 mL/min. According to the FDA-approved label, corresponding doses are 80 mg PO equals 75 mg IV, 120 mg PO equals 112.5 mg IV, and 160 mg PO equals 150 mg IV.
Two placebo-controlled trials that studied patients in sinus rhythm who had at least one documented prior episode of AF found sotalol to be safe and effective at doses ranging from 80 to 160 mg twice daily. However, clinicians should be aware of the fact that QT monitoring is essential during treatment with sotalol; an absolute QT greater than 500 ms, or a QT prolongation greater than 60 ms regardless of absolute value, excessively increases the risk of TdP.
A randomized double-blind study found that a 5-minute IV infusion of sotalol 100 mg was more effective than a 5-minute infusion of 100 mg lidocaine in patients with spontaneous, hemodynamically stable, sustained monomorphic VT within a hospital setting. Another study in 109 patients with a history of spontaneous and inducible sustained VT showed that the infusion of 1.5 mg/kg of sotalol over 5 minutes or less was effective.
Modification of Dosage in Disease States
Because sotalol is mainly eliminated unchanged in the urine, the dosage must be adjusted for altered renal function. For patients with a CLCR greater than 60 mL/min, the usual dosing interval is every 12 hours. If the CLCR is between 30 and 60 mL/min, the recommended interval between doses is 24 hours. For patients with CLCR between 10 and 30 mL/min, the interval should be every 36 to 48 hours, or the usual dose may be halved and given every 24 hours. The dosage for patients with CLCR less than 10 mL/min should be individualized. Because of the increased risk of proarrhythmia and CHF, patients with reduced cardiac output should be given lower doses and should be monitored carefully.
Adverse Reactions
A major concern with sotalol treatment has been the occurrence of TdP. As of the fourth quarter of 2011, a total of 244 reports of TdP had been reported to the FDA’s Adverse Events Reporting System (AERS). Clearly, hypokalemia, hypomagnesemia, and bradycardia are predisposing factors for the development of this arrhythmia during sotalol therapy, as they are with quinidine, disopyramide, and procainamide. It is more common in females, in patients with CHF, and in those with a history of sustained VT. The incidence of TdP should be minimized by careful screening and consideration of predisposing factors such as female gender, bradycardia, baseline prolongation of the QT interval, and electrolyte disturbances, especially hypokalemia; careful dose escalation, beginning at 160 mg/day; and limitation of the maximum QT interval prolongation to less than 500 ms. Sotalol should not be administered with other drugs that prolong the QT interval. A list of such drugs can be found at www.QTdrugs.org .
The incidence of new or worsened CHF is about 3%. This may be attenuated because of the increased inotropy produced by its action to prolong repolarization. Other side effects typical of β-blockers are to be expected, including bronchospasm in asthmatic patients, masking the signs and symptoms of hypoglycemia in diabetic patients, and catecholamine hypersensitivity withdrawal syndrome.
Drug Interactions
Concomitant use of sotalol with agents that prolong repolarization has the potential to increase the likelihood of TdP. No pharmacokinetic interactions have been seen with sotalol and/or warfarin, digoxin, cholestyramine, or hydrochlorothiazide. Because of the β-blocking actions of sotalol, it is likely that there would be increased pharmacologic effect, if the drug were to be combined with amiodarone, calcium channel blockers, antihypertensive agents, clonidine, or antiarrhythmic agents.
Amiodarone
Clinical Applications
Amiodarone has been reported to have efficacy in a wide range of arrhythmias. Numerous trials in the literature describe the efficacy of amiodarone in the conversion and slowing of AF, AV nodal reentrant tachycardia, and tachycardias associated with the Wolff-Parkinson-White syndrome.
After the results of CAST, antiarrhythmic drugs were examined for their effects on mortality rate. The Veteran’s Administration trial, Congestive Heart Failure Survival Trial of Antiarrhythmic Therapy (CHF STAT), examined the effects of amiodarone on total mortality in patients with a history of CHF, more than 10 premature ventricular contractions per hour on ambulatory monitoring, and an ejection fraction less than 40%. The study found no difference in the placebo and amiodarone arms. Two other major trials evaluated amiodarone in patients with recent MI. The Canadian Myocardial Infarction Amiodarone Trial (CAMIAT) and the European Myocardial Infarction Amiodarone Trial (EMIAT) were completed in the last decade. The results of these trials were mixed in that neither found amiodarone to reduce overall mortality, but the Canadian trial reported a reduced incidence of ventricular fibrillation or arrhythmic death among survivors of MI with ventricular ectopy. Importantly, no increase in mortality was noted, as has been seen with other antiarrhythmic drugs. Meta-analyses of the many trials with amiodarone have generally confirmed a slight reduction in mortality rate in cardiac patients. The NIH-sponsored Antiarrhythmics Versus Implanted Devices (AVID) trial found that the devices were superior to amiodarone in reducing mortality in patients who had been resuscitated from sudden death or in those who had sustained VT.
In 1993, an IV formulation of amiodarone became available in the United States. Three completed controlled trials demonstrated the value of amiodarone in patients with recurrent life-threatening VT or fibrillation, and a comparison of three dosages (125, 500, and 1000 mg/day) found that the recurrence of arrhythmia was lowest at the higher dosages. Hypotension was the major side effect seen, but it occurred equally, about 26%, in all groups. The second study in a similar group of patients was a comparison of bretylium to two doses of amiodarone. The arrhythmia event rate for the first 48 hours of therapy was equivalent for the high dose of amiodarone and bretylium, and both were more effective than the low dose of amiodarone. Hypotension was common in all groups but was significantly higher in the bretylium group. Amiodarone was approved for IV therapy of ventricular arrhythmia by the FDA in 1998. Although not yet approved in labeling, a study found IV amiodarone effective for prevention of postoperative AF.
Mechanism of Action
Amiodarone is an iodinated benzofuran that has structural similarity to thyroxine and procainamide and was originally developed as an antianginal agent. It was incidentally noted to suppress a wide variety of ventricular and supraventricular arrhythmias. It has been assumed that this efficacy is caused by its prolongation of refractoriness and APD in myocardial tissue (Vaughan Williams class III antiarrhythmic activity), although amiodarone has been found to have many diverse pharmacologic actions. The actions responsible for its high degree of antiarrhythmic efficacy remain unidentified.
In intracellular recordings of rabbit cardiac myocytes, amiodarone prolongs APD and increases refractoriness of both atrial and ventricular myocardium and of Purkinje fibers and sinus and AV nodal tissues. Amiodarone decreases phase 3 depolarization of myocardial cells, blocks sodium channels that are in the inactivated state, slows phase 4 depolarization of the sinus node, and slows conduction through the AV node. The electrophysiologic actions of the major metabolite of amiodarone, desethyl-amiodarone (DEA), differ from those of amiodarone, with the metabolite having greater effects on conduction because of its effects on sodium channels and therefore on conduction. Intracoronary injection of amiodarone has shown little cardiac effect compared with DEA’s ability to prolong cardiac refractoriness.
Electrophysiologic changes in humans depend on the route of administration and the duration of therapy. Following acute IV amiodarone administration, prolongation of the AH interval and an increase in the refractory periods of the AV node and bypass tracts are seen, but this may be due to the presence of the solubilizing agent, polysorbate 80 (Tween 80), in the IV formulation. No acute changes occur in either sinus rate or atrial or ventricular refractoriness, whereas these are prolonged during chronic oral therapy. Chronic amiodarone therapy also prolongs the AH and HV intervals and the PR and QT intervals of the surface ECG. Conflicting reports on the time course of these changes are found, and it is not clear how they might relate to antiarrhythmic efficacy.
Changes in APD and refractoriness are seen in hypothyroidism similar to changes resulting from oral amiodarone therapy. These changes can be prevented in animals by coadministration of thyroid hormone with amiodarone, leading to the conclusion that amiodarone’s antiarrhythmic efficacy is attributed to production of “cardiac hypothyroidism.” This is supported by the observation that amiodarone’s major metabolite causes noncompetitive inhibition of thyroid hormone binding to nuclear receptors. On the other hand, amiodarone also causes noncompetitive blockade of α- and β-receptors, muscarinic receptors, and both calcium and sodium channel blockade, any combination of which may contribute to its antiarrhythmic efficacy.
Clinical Pharmacology
Amiodarone is a highly lipid-soluble compound with extremely variable and complex pharmacokinetics. It is slowly absorbed from the gastrointestinal tract, and bioavailability varies over a fourfold range. Amiodarone is extensively metabolized to DEA by CYP3A4 and CYP2C8, and little if any is excreted unchanged in the urine. Concentrations of DEA in plasma vary from 0.4 to 2.0 times that of amiodarone during long-term therapy. This metabolite has antiarrhythmic potency equal to or greater than amiodarone in in vitro and in animal models. Amiodarone is rapidly concentrated in some tissues, including myocardium, but it accumulates more slowly in others, such as adipose tissue. It redistributes out of myocardial tissue while still accumulating in adipose and other tissues. Until all tissues are saturated, rapid redistribution out of the myocardium may be responsible for early recurrence of arrhythmias after discontinuation of therapy or rapid reduction of dosage. Because of drug accumulation in tissues, the volume of distribution for amiodarone is very large, 20 to 200 L/kg. After IV administration, the measured half-life in plasma is from 4.8 to 68.2 hours, with tissue uptake being the primary factor responsible for the decline in plasma concentration. As tissues become saturated, however, the decline in plasma levels is slow, reflecting mainly elimination and slow redistribution of the drug out of adipose and muscle tissues. This leads to slow and extremely variable elimination from plasma, with half-lives ranging from 13 to 103 days at steady state. It is also possible that amiodarone inhibits its own elimination after chronic therapy, contributing to the differences between half-life early in therapy to that after prolonged therapy.
Dosage and Administration
Without a loading-dose regimen, amiodarone requires several weeks to months before producing its antiarrhythmic action; however, large IV doses or oral loading doses can hasten the onset of therapeutic effects. From small prospective studies, loading doses have varied from 600 to 1400 mg/day for 2 to 21 days. Recent large clinical trials have used a lower loading dose of 600 to 800 mg/day for 14 days. Because of relatively rapid redistribution out of myocardial tissue, the dosage should be tapered over a period of several weeks. The usual maintenance dose varies from 200 to 600 mg/day, and because of the severe nature of adverse reactions, the lowest effective dose should be prescribed. Patients with supraventricular arrhythmias may respond to lower doses than those with ventricular arrhythmias, but there are many exceptions. Because of the variable pharmacokinetics and oral bioavailability, generalizations such as this may be unreliable. Some patients with extensive absorption (80% to 90% bioavailability) of even low doses may have the same drug exposure as a person with limited bioavailability given a high dose.
For IV administration, the manufacturer recommends a three-phase infusion over the first 24 hours: 150 mg over 10 minutes, followed by 360 mg over the next 6 hours, followed by 0.5 mg/min. The drug can be continued at this rate, but monitoring of plasma concentrations is recommended. An additional 150 mg can be infused over 10 minutes for patients who continue to have recurrent VT or fibrillation or those whose arrhythmia recurs during downward titration of the infusion. Concentrations of drug greater than 3 mg/mL should be infused through a central catheter to prevent phlebitis. Also, the surfactant properties of the drug alter the size of a drop of infusate, and pumps that count drops will give approximately 30% less drug than intended.
Amiodarone concentrations are usually between 1 and 2 µg/mL during effective oral therapy. Similar concentrations of DEA accumulate during therapy, and although this is unproven, it is likely to contribute to antiarrhythmic efficacy. Because of extensive overlap between the range of concentrations required for arrhythmia suppression and those associated with toxicity, monitoring of plasma concentrations is of limited value. Levels of amiodarone above 3 to 4 µg/mL for prolonged periods of time are clearly associated with a higher incidence of adverse effects.
Modification of Dosage in Disease States
Long-term oral therapy with amiodarone appears to be well tolerated hemodynamically in patients with CHF. In the Veteran’s Administration trial discussed above, amiodarone failed to prolong life for CHF patients with arrhythmias but was associated with improved ventricular function as measured by radionuclide ejection fraction.
Adverse Reactions
IV amiodarone at dosages greater than 5 mg/kg decreases cardiac contractility and peripheral vascular resistance and produces severe hypotension in some instances. Because oral administration at usual dosages improves myocardial contractility, some of this effect, like the electrophysiologic effects described earlier, may be due to the effects of polysorbate 80 or benzyl alcohol.
The safety of amiodarone is controversial. Early reports found it to be very well tolerated and described it as the “ideal” antiarrhythmic drug. Some studies continue to find that it is relatively safe and effective, even in the treatment of arrhythmias in children. The early experience with amiodarone in the United States, with a very high incidence of intolerable and sometimes lethal reactions, may have been the result of high dosages required for control of life-threatening arrhythmias. Under less urgent conditions, lower dosages are given and are much better tolerated. Determination of the incidence of adverse reactions is difficult because of highly variable dosages and durations of treatment.
The most serious adverse reaction is lethal interstitial pneumonitis, which may be more frequent in patients with preexisting lung disease. Monitoring is essential, because the pneumonitis is reversible if detected early. A chest radiograph every 3 months may be useful, but serial pulmonary function tests are of little value for follow-up. Hyperthyroidism or hypothyroidism occurs in about 4% of patients treated long-term. Accumulation of corneal microdeposits is almost uniform during long-term therapy and in many cases can progress to the point of interfering with vision. Some white patients develop a slate gray or bluish discoloration of sun-exposed areas of the skin. Many also report photosensitivity, which can sometimes be prevented or alleviated with sunscreens and garments, and 30% or more patients have abnormally elevated serum hepatic enzyme levels, and progression to jaundice and cirrhosis has been reported in some of these patients. Serial laboratory tests to screen for amiodarone toxicity can be costly and generally are of limited value; however, it is wise to obtain a reliable assessment of baseline tests, including complete blood count, blood chemistry, tests of thyroid and pulmonary function, a slit-lamp examination, and measurement of blood levels of other drugs whenever possible due to frequent drug interactions.
Drug Interactions
Amiodarone interferes with the clearance of many drugs. This may involve the formation of a metabolically inactive CYP Fe(II)–metabolite complex, which has been described in animals treated with amiodarone and may explain the reduced metabolism and unexpected accumulation of warfarin, quinidine, procainamide, disopyramide, mexiletine, and propafenone and the resulting bleeding, heart block, or TdP. It does not, however, explain interaction with drugs eliminated predominantly by the kidneys, such as digoxin. Here the effect is mediated by inhibition of P-glycoprotein. The elimination of other drugs may be impaired by amiodarone, and the lowest effective dosage should be sought.
Ibutilide
Clinical Applications
In 1995, ibutilide was given FDA approval for the rapid conversion of recent-onset AF or atrial flutter, and testing in other arrhythmias and in patients with AF or atrial flutter of long duration (>90 days) was completed. Ibutilide should not be given to patients who have hypokalemia, hypomagnesemia, or QTc prolongation at baseline greater than 440 ms. In placebo-controlled studies summarized in the manufacturer’s labeling, the placebo conversion rate for AF or atrial flutter was approximately 2%. Ibutilide terminated the arrhythmia in approximately 43% to 48% of patients treated with 1 mg followed by either 0.5 or 1 mg. Approximately 20% of patients responded to the first infusion, and approximately 25% of those who did not respond to the first infusion responded to the second infusion. Response usually occurred at 20 to 30 minutes but ranged from 5 to 88 minutes after infusion. The response in patients with AF and atrial flutter was not significantly different in the early trials performed. However, in patients with postoperative arrhythmias, a greater response was seen in patients with atrial flutter, with a conversion rate of 53% to 72% compared with 20% with placebo. Ibutilide may have value in conversion of AF in patients with Wolff-Parkinson-White syndrome.
Mechanism of Action
Ibutilide is a remarkably potent methanesulfonamide analogue of sotalol that prolongs cardiac APD and has class III action (i.e., it prolongs cardiac refractoriness and APD), and it appears that ibutilide has multiple mechanisms of action. The manufacturer’s data indicate that the drug’s class III action is due to an increase in inward sodium current, as observed in guinea pig ventricular myocytes at 10 −7 mol/L concentrations. They observed that higher concentrations (10 −5 mol/L) increase an outward potassium current to shorten APD. Other investigators reported that, as has been seen with dofetilide, sotalol, and other methanesulfonamides, 10 −8 mol/L concentrations of ibutilide block the rapid component of the delayed rectifier potassium current, I Kr , in mouse and human cardiac cells.
Clinical Pharmacology
Ibutilide is only available for IV administration. When given over 10 minutes, it distributes rapidly in a multiexponential fashion, and the relevant component has a half-life from 2 to 12 hours (mean, 6 hours). The plasma concentration and pharmacokinetics are highly variable, and dosing is recommended on the basis of weight. The drug is mainly eliminated by oxidative hepatic metabolism with sequential β-oxidation to eight metabolites, systemic clearance is rapid (approximately 29 mL/min/kg), and protein binding is about 40%. According to animal models, only one metabolite possesses class III antiarrhythmic effects similar to those of ibutilide; however, the plasma concentration of this metabolite is less than 10% of that of ibutilide. Because formal drug interaction studies have not been performed, it is not possible to anticipate which enzymes are likely responsible for its biotransformation.
Dosage and Administration
Ibutilide is administered undiluted or diluted in saline as an infusion over 10 minutes. The recommended dose for a patient heavier than 60 kg is 1 mg, and a dose of 0.01 mg/kg is recommended for a patient weighing less than 60 kg. For patients whose arrhythmias have not converted by 10 minutes after completion of the first dose, a second dose of equal magnitude can be administered. Because conversion of arrhythmias is usually associated with peak levels, slower infusion rates are not likely to be as effective.
Because of ibutilide’s significant risk of TdP, it is essential that patients who receive this drug be treated in a monitored environment for at least 4 hours after administration. The FDA-approved labeling recommends that skilled personnel, facilities, and medication for defibrillation or resuscitation be readily available. A retrospective analysis indicated that pretreatment with magnesium sulfate can reduce the estimated 3% to 4% incidence of TdP by approximately 20% to 30%. A subsequent controlled trial found that two 5-g doses of magnesium sulfate reduced the incidence of TdP associated with ibutilide administration.
Modification of Dosage in Disease States
Specific studies with HF and renal or hepatic disease have not been conducted. Patients with severe LV dysfunction have a higher risk of developing ventricular arrhythmias, including TdP. Because the duration of drug effect is determined by distribution, it is very possible that patients with severe CHF will have decreased volumes of distribution and hence an exaggerated and prolonged duration of effect.
According to the manufacturer, it is unlikely that dosage adjustments are necessary in patients with impaired renal or hepatic function, based on the considerations that ibutilide is indicated for rapid IV therapy (duration of 30 minutes or less) and is dosed to a well-defined goal (termination of arrhythmia) and that drug distribution appears to be one of the primary mechanisms responsible for termination of the pharmacologic effect. Nonetheless, patients with abnormal hepatic function should be monitored for longer than the recommended 4-hour interval afterward.
Adverse Reactions
The most serious adverse reaction to ibutilide is TdP; however, only 586 patients participated in trials before marketing, and patients with a QT C greater than 440 ms or potassium concentrations less than 4 mEq/L were excluded. Despite these precautions, the incidence of sustained polymorphic VT requiring cardioversion was 1.7%. Another 2.7% developed nonsustained polymorphic VT, 4.9% had nonsustained monomorphic VT, 1.5% had AV block, and 1.9% had bundle branch block. The risk of polymorphic VT in some studies was highest in patients who were female and in those who had evidence of reduced ventricular performance. The incidence of these adverse effects may well be higher in general clinical use, in which electrolyte disorders and concomitant therapies may be more common. As of the fourth quarter of 2011, a total of 114 reports of TdP were reported to be associated with ibutilide in the FDA’s AERS database. Bradycardia and multiple episodes of sinus arrest have also been reported, along with a single case of acute renal failure.
Drug Interactions
No specific drug interaction studies have been performed. Concomitant β-receptor or calcium channel antagonists do not apparently interact, although data are limited. The manufacturer’s labeling warns against combining ibutilide with other drugs that prolong the QT interval (see www.QTdrugs.org ). During ibutilide’s development, these drugs were discontinued for at least five half-lives prior to administration of ibutilide and were not allowed until at least 4 hours after administration.
Dofetilide
Clinical Applications
Dofetilide was approved and marketed in 2000 for oral therapy of AF and atrial flutter. In controlled trials of approximately 1000 patients, about 30% of those with AF who were given a dosage of 500 µg twice daily converted to normal sinus rhythm compared with 6% in the control group treated with sotalol and 1% of patients given placebo. Prevention of recurrence was demonstrated with 62% to 71% remaining in sinus rhythm after 6 months compared with 59% for sotalol and 26% to 37% for placebo (S. Singh, personal communication). A large mortality trial, the Danish Investigators of Arrhythmia and Mortality on Dofetilide (DiAMOND) trial, examined the effects of dofetilide on mortality rate and AF in 1518 patients with reduced ejection fraction and symptoms of HF. A decrease in the incidence of hospitalization for HF was observed. Although dofetilide’s antiarrhythmic efficacy was confirmed in the lower incidence of AF, a positive effect on mortality was not observed. This is in stark contrast to the previously observed increases in mortality rate with sodium channel blockers (CAST and CAST-II) and with d-sotalol (SWORD). A caveat to this safety finding was the potentially important role of extensive screening and monitoring for potential harm. Even with these efforts, 3.3% of patients in this trial developed TdP. Because of the risk of TdP, the manufacturer requires physicians to receive special training before prescribing dofetilide, and the FDA has required that labeling include a boxed warning that therapy should be initiated in the hospital with continuous ECG monitoring for at least 3 days.
Mechanism of Action
Dofetilide is one of the most potent I KR blockers ever synthesized. Perhaps an additional advantage is the twofold greater ability to prolong APD in atrial compared with ventricular tissue. It does not appear to depress cardiac function at usual dosages even in patients with reduced ejection fraction.
Clinical Pharmacology
Dofetilide is well absorbed after oral administration and is partially metabolized by CYP3A4 to inactive metabolites, and it is excreted predominantly in urine. In most patients, the elimination half-life ranges from 8 to 10 hours but is prolonged, and clearance is reduced, in patients with renal failure. Dofetilide is susceptible to several drug interactions, because it is metabolized by CYP3A4 (see below). It is very likely that some of these interactions increase the risk of TdP.
Dosage and Administration
The recommended dosage of dofetilide is 500 µg twice daily. Lower dosages are recommended for patients who develop excessive QT-interval prolongation on 500 µg twice daily. In the largest clinical trial, “excessive” was defined as greater than 550 ms or more than 20% longer than baseline.
Modification of Dosage in Disease States
Dosage should be reduced in patients with renal disease (250 µg twice daily for CLCR 60 to 40 mL/min and 250 µg four times a day for CLCR 40 to 20 mL/min). Data are not available for adjustment of dosage in patients with liver disease, and it is not clear whether the greater risk of TdP in women is influenced by a pharmacokinetic difference between the sexes.
Adverse Drug Reactions
The major adverse effect of dofetilide is TdP, and the overall incidence during clinical development was 0.9%. In the DiAMOND trial, 3.3% of patients with a history of HF developed TdP.
Drug Interactions
Concomitant administration of dofetilide with verapamil, ketoconazole, or cimetidine—but not ranitidine—results in increased plasma concentrations of dofetilide, especially in patients with reduced renal function. Because it is known to be a substrate for CYP3A4, there may be other potential interactions with erythromycin, other macrolides, or antifungals. No interactions have been seen between dofetilide and digoxin or warfarin.
Adenosine
Clinical Applications
Adenosine is very effective for the acute conversion of paroxysmal supraventricular tachycardia (PSVT) caused by reentry involving accessory bypass pathways. At a dose of 6 mg, 60% of patients respond, and an additional 32% respond when given a 12-mg dose. Because of the fleeting and relatively selective action of adenosine on the AV node, some have suggested that it be used as a diagnostic tool in patients with narrow and wide complex tachycardia. However, when possible, it is preferable to make the correct diagnosis before giving any drugs because of their risk of adverse effects.
Mechanism of Action
Adenosine is a nucleoside formed in the body by serial dephosphorylation of adenosine triphosphate (ATP), from cyclic adenosine monophosphate or from hydrolysis of S -adenosylhomocysteine. It is formed both inside and outside the cell, and its actions are rapidly terminated by active transport into cells, followed by metabolism. The actions of adenosine are highly dependent on the rate and route of administration. A rapid IV injection into a central venous line is thought to activate carotid body chemoreceptors and usually produces an initial increase in blood pressure of 10 to 15 mm Hg, followed by a small and transient decrease. These reflexes are attenuated during surgery, and in this setting, adenosine decreases peripheral vascular resistance, increases cardiac output, and increases heart rate moderately. Bolus injections also produce biphasic effects on heart rate. Approximately 20 seconds after injection, sinus bradycardia occurs for 10 to 15 seconds, followed by sinus tachycardia thought to be due to chemoreceptor activation. Activation of the carotid chemoreceptors stimulates respiration and causes secondary activation of pulmonary stretch receptors. Adenosine has a direct effect of slowing AV nodal conduction, which can result in transient AV block. Although adenosine has no direct effect on the His-Purkinje system, it does attenuate the effects of catecholamine stimulation and, in patients with heart block, it can block acceleration of the ventricular escape rate by isoproterenol. Adenosine usually has no effect on anterograde or retrograde accessory pathway conduction. Pathways that demonstrate decremental conduction often respond to adenosine, probably because they are partially depolarized and can be hyperpolarized by adenosine. Slow injections into a peripheral line often produce no clinical benefit or changes in blood pressure or heart rate.
The development of synthetic agonists and antagonists of adenosine receptors has made possible the subclassification of A 1 and A 2 receptor subtypes. The A 1 receptors are present in myocardial cells and mediate the negative inotropic, dromotropic, and chronotropic actions of adenosine. The A 2 receptors are present in the endothelium and vascular smooth muscle cells and cause coronary vasodilation when activated.
The efficacy of adenosine in PSVT is most likely a result of actions in atrial myocardium and in the AV node, such as 1) hyperpolarization of sinoatrial (SA) node cells and slowing of firing rate, 2) shortening of the action potential duration of atrial cells, and 3) depression of conduction velocity in the AV node. These actions are due to activation of A 1 adenosine receptor subtypes, which leads to activation of cyclic adenosine monophosphate (cAMP)–independent, acetylcholine/adenosine-regulated potassium current, I ACh,Ado .
Clinical Pharmacology
After IV injection, adenosine is rapidly transported into red blood cells and endothelial cells. Half-life of elimination has ranged from 1.5 to 10 seconds, and the drug is rapidly metabolized in the plasma and in cells to form inosine and AMP. Maximal pharmacologic effects are seen within 30 seconds after injection into a peripheral IV line but occur within 10 to 20 seconds when given into a central line.
Dosage and Administration
Adenosine should be injected intravenously into a proximal tubing site and flushed quickly with 20 mL saline. For adults, the initial dose is 6 mg injected over 1 to 2 seconds. If the arrhythmia persists, a 12-mg dose can be injected 1 to 2 minutes later. This can be repeated, but doses larger than 12 mg are not recommended by the manufacturer. A dosage regimen based on body weight has been proposed, with an initial dose of 50 µg/kg incremented by 50 µg/kg until the PSVT is terminated, or side effects become intolerable. Higher doses may be required for patients who have received caffeine or theophylline because of their antagonistic effects at A 1 receptors. Lower doses are recommended for patients receiving dipyridamole or carbamazepine.
Modification of Dosage in Disease States
Although the pharmacokinetics of adenosine are unlikely to be altered in patients with renal or hepatic disease, these patients often have electrolyte imbalances that could alter the clinical response. Although patients with CHF have not been reported to respond abnormally, heart transplant recipients appear to require one third to one fifth of the usual dose because of denervation hypersensitivity.
Adverse Reactions
Adenosine is contraindicated in patients with sick sinus syndrome or second- or third-degree heart block, unless the patient has a functioning artificial pacemaker. Because of the rapid clearance of adenosine, side effects such as facial flushing, dyspnea, or chest pressure last less than 60 seconds. Although intrapulmonary administration of adenosine has precipitated bronchospasm in asthmatic patients, this has not been reported with IV administration. Other less frequent side effects include nausea, lightheadedness, headache, sweating, palpitations, hypotension, and blurred vision. IV theophylline, which has been recommended to reverse the effects of adenosine, should be prepared and ready for injection in high-risk patients.
Drug Interactions
Several proven interactions can increase or decrease the activity of adenosine. Dipyridamole pretreatment increases the potency of adenosine, probably because it blocks cellular uptake of adenosine. On the other hand, caffeine and theophylline antagonize the actions of adenosine, and the manufacturer cautions that carbamazepine may potentiate the actions of adenosine.
Dronedarone
Clinical Applications
Dronedarone was approved by the FDA in July 2009 to reduce the risk of hospitalization for persistent or recurring AF and atrial flutter. Dronedarone is an antiarrhythmic agent developed with the aim of achieving an efficacy similar to that of amiodarone with an improved safety and tolerability profile. A comparison among dronedarone, amiodarone, and placebo in the trial to evaluate the Effiacy and Safety of Dronedarone versus Amiodarone for the Maintenance of Sinus Rhythm in Patients with Atrial Fibrillation (DIONYSOS) employed a primary composite efficacy endpoint of recurrence of AF that included unsuccessful electrical cardioversion, no spontaneous conversion, and no electrical cardioversion or premature study discontinuation and a main safety endpoint that aggregated the occurrence of events specific to the eyes, skin, and thyroid and hepatic, pulmonary, neurologic, and gastrointestinal systems, or premature study drug discontinuation following an adverse event. Results showed that the composite efficacy endpoint was met in 75.1% and 58.8% with dronedarone and amiodarone respectively at 12 months (hazard ratio [HR], 1.59; 95% confidence interval [CI], 1.28 to 1.98; P < .0001), driven mainly by AF recurrence with dronedarone compared with amiodarone (63.5% vs. 42.0%). AF recurrence after successful cardioversion was 36.5% and 24.3% with dronedarone and amiodarone, respectively. Premature drug discontinuation tended to be less frequent with dronedarone (10.4% vs 13.3%). The frequency of the main safety endpoint was 39.3% and 44.5% with dronedarone and amiodarone, respectively, at 12 months (HR, 0.80; 95% CI, 0.60 to 1.07; P = .129). These results were driven mainly by fewer thyroid, neurologic, skin, and ocular events in the dronedarone group.
The dronedarone label contains a boxed warning that contraindicates its use in patients with New York Heart Association (NYHA) class IV heart failure or NYHA class II to III heart failure with a recent decompensation requiring hospitalization or referral to a specialized HF clinic. This warning is due to results from the European Trial of Dronedarone in Moderate to Severe Congestive Heart Failure (ANDROMEDA), which led to the trial’s premature discontinuation. As opposed to previous dronedarone trials, ANDROMEDA enrolled high-risk patients with symptomatic HF and severe LV systolic dysfunction. The twofold increase in mortality seen in ANDROMEDA was predominately owed to worsening HF. Because of modifications in the chemical structure of dronedarone—that is, removal of the iodine moiety and addition of a methanesulfonyl group—dronedarone has lower lipophilicity, thus resulting in a shorter half-life of 13 to 19 hours, compared with 58 days for amiodarone, and lower tissue accumulation compared with amiodarone. These characteristics of the dronedarone molecule are postulated to account for the proposed improved safety profile seen with dronedarone; however, trials lasting longer than 12 months have not been conducted. Dronedarone is indicated to reduce the risk of CV hospitalization in patients with paroxysmal or persistent AF who are in sinus rhythm or will be cardioverted and who have had a recent episode of AF and have associated CV risk factors: age greater than 70 years, hypertension, diabetes, prior cerebrovascular accident, left atrial diameter of 50 mm or more, or LV ejection fraction less than 40%. (See Chapter 20 for a discussion of the PALLAS trial.)
Mechanism of Action
Dronedarone is a benzofuran analogue of amiodarone. The exact mechanism of action of dronedarone is yet unknown, but like amiodarone, dronedarone has complex electrophysical effects belonging to all four Vaughan Williams classes. As a drug with class III effects, dronedarone prolongs the cardiac action potential and refractory periods by inhibiting the potassium currents I Kr , I K1 , I KACh , and I sus . It also inhibits sodium channels, which results in a decreased slope of the depolarization phase of the action potential, a class IB effect, and also inhibits the slow L-type calcium channels, a class IV effect. Additionally, dronedarone has shown antiadrenergic effects (class II). In healthy subjects, dronedarone was found to significantly prolong the R-R and QT interval in a dose-dependent manner. The removal of the iodine moiety described above was also aimed at reducing the risk of thyroid function abnormalities known to occur with amiodarone therapy. Animal studies indicate dronedarone does not affect circulating plasma thyroid hormones.
Clinical Pharmacology
Dronedarone is administered orally. Dronedarone and its metabolites are more than 98% bound to plasma proteins, mainly albumin, and this protein binding is not saturable. Dronedarone is extensively metabolized, mainly involving the CYP3A4 isoenzyme. The main active metabolite, desbutyl-dronedarone, is 0.1 to 0.3 times as potent as dronedarone. Approximately 30 other uncharacterized metabolites of dronedarone also exist. About 6% of radiolabeled dronedarone is excreted in urine, and 84% is excreted in feces, mainly as metabolites. The elimination half-life of dronedarone is 13 to 19 hours.
Dronedarone possesses electrophysiologic properties of all four Vaughan Williams classes. Dronedarone was evaluated in healthy volunteers receiving repeated oral doses of up to 1600 mg once daily or 800 mg twice daily for 14 days and 1600 mg twice daily for 10 days. A dose-dependent increase in the PR interval of 5 ms occurred in the group that received 400 mg twice daily, and an increase of up to 50 ms occurred in the 1600 mg twice-daily group. A similar dose-related effect occurred in the QT interval with an increase of 10 ms in the 400 mg twice daily group and an increase of up to 25 ms in the 1600 mg twice daily group.
Individualization of Dosage
Two trials, European Trial in Atrial Fibrillation Patients Receiving Dronedarone for the Maintenance of Sinus Rhythm (EURIDIS) and the American-Australian-African Trial with Dronedarone in Atrial Fibrillation Patients for the Maintenance of Sinus Rhythm (ADONIS), were identical multicenter, double-blind, randomized trials to evaluate the efficacy of dronedarone (400 mg twice daily; n = 828) vs. placebo (n = 409) for the maintenance of sinus rhythm in AF or atrial flutter over a 12-month study period. The median time to first recurrence of arrhythmia was significantly longer in the dronedarone group compared with the placebo group (EURIDIS: 96 vs. 41 days, P = .01; ADONIS: 158 vs. 59 days, P = .002). In addition, the rates of recurrence of AF were significantly lower for the dronedarone group compared with placebo for both trials (EURIDIS: 67.1% vs. 77.5%, respectively; ADONIS: 61.1% vs. 72.8%, respectively).
A Placebo-Controlled, Double-Blind, Parallel-Arm Trial to Assess the Efficacy of Dronedarone 400 mg bid for the Prevention of Cardiovascular Hospitalization or Death from any Cause in Patients with Atrial Fibrillation/Atrial Flutter (ATHENA) involved 4628 patients with AF or atrial flutter. The trial included patients with stable HF but excluded patients who were clinically decompensated. The primary endpoint was time to first hospitalization owing to cardiovascular (CV) events or death from any cause. CV hospitalization or death occurred in 917 patients (39.4%) in the placebo group and in 734 patients (31.9%) in the dronedarone group. There were 116 deaths (5%) in the dronedarone group and 139 deaths (6%) in the placebo group. Of these deaths, 63 (2.7%) were from CV causes—nonarrhythmic cardiac causes, cardiac arrhythmia, noncardiac vascular causes—in the dronedarone group, and 90 (3.9%) were from CV events in the placebo group, which was due to the difference in cardiac arrhythmia seen between the dronedarone and placebo groups (26 [1.1%] vs. 48 [2.1%], respectively).
Modification of Dosage in Disease States
Limited data are available, but according to the FDA-approved label, dosage adjustments are not needed in mild to moderate hepatic impairment. However, dronedarone is considered contraindicated in patients with severe liver impairment. Specific guidelines are not available for dosage adjustments in renal impairment.
Adverse Reactions
An increase in serum creatinine concentration of 10% or more after 5 days of treatment initiation was seen in 51% of patients receiving dronedarone compared with 21% of those receiving placebo.
CV effects of dronedarone are difficult to differentiate from extensions of its normal pharmacologic activity. In the ATHENA outcomes trial, bradycardia was reported in 3.5% of patients in the dronedarone group compared with 1.2% of patients taking placebo ( P < .001). In combined data from EURIDIS and ADONIS, bradycardia was reported in 2.7% of patients in the dronedarone group compared with 2% in the placebo group ( P = .56), and baseline heart rate was reduced in the dronedarone group by 6.8% ( P < .001).
According to the manufacturer, QT prolongation, defined as greater than 450 ms for males and greater than 470 ms for females, occurred in 28% of patients receiving dronedarone in clinical trials compared with 19% of those receiving placebo. One patient in the ATHENA trial developed TdP while receiving dronedarone. Combined data from EURIDIS and ADONIS show that when compared with placebo, dronedarone prolonged the QT C interval by 9 ms ( P < .001) with no significant effects on QRS duration. As of the fourth quarter of 2011, a total of 47 reports of TdP associated with dronedarone were filed in the FDA’s registry of adverse events (AERS).
Dronedarone is associated with worsening HF and increased mortality rate in patients with NYHA class IV heart failure or NYHA class II to III heart failure with a recent decompensation requiring hospitalization or referral to a specialized HF clinic, and these conditions are considered absolute contraindications for dronedarone in FDA-approved labeling.
Additional contraindications include use in pregnant or nursing women; the coadministration of strong CYP3A4 inhibitors such as ketoconazole, clarithromycin, or certain antiretrovirals such as nelfinavir or ritonavir; AV block of second or third degree; sick sinus syndrome, except when used in conjunction with pacing; a heart rate less than 50 beats/min; QT C of 500 ms or more; and PR greater than 280 ms.
The FDA-approved label also includes a warning on the potential for hepatotoxicity that includes life-threatening liver failure. Several reports of hepatocellular liver injury and hepatic failure have been reported in patients treated with dronedarone. Two cases of acute hepatic failure requiring transplantation occurred in patients with previously normal hepatic serum enzymes. In both cases, the patients were female and approximately 70 years of age, and hepatic failure occurred within 6 months of therapy. In the first case, the patient presented with jaundice, coagulopathy, elevated hepatic enzymes, and hyperbilirubinemia, which then progressed to hepatic encephalopathy. In the second case, the patient presented with weakness, abdominal pain, coagulopathy, elevated hepatic enzymes, and hyperbilirubinemia. In both cases, alternative etiology for liver failure was not identified, and extensive hepatic necrosis was seen in the explanted liver. Although it is not known whether monitoring of hepatic serum enzymes will prevent the development of severe hepatic injury, clinicians are advised to consider obtaining periodic hepatic serum enzymes in patients treated with dronedarone, especially during the first 6 months of treatment. Dronedarone treatment should be discontinued immediately if hepatic injury is suspected, and serum liver enzymes and bilirubin concentrations should be obtained. Dronedarone should not be restarted in patients who experience hepatic injury during treatment without another explanation for the injury.
Drug Interactions
Dronedarone is both a substrate for and an inhibitor of CYP3A4, and it is a CYP2D6 inhibitor that can also inhibit P-glycoprotein transport. Therefore caution is advised in the setting of other drugs metabolized by these hepatic CYP450 systems. There is an almost twofold increase in digoxin concentrations and a twofold to fourfold increase in simvastatin concentrations when these agents are used concomitantly with dronedarone. β-Blockers and calcium channel blockers (diltiazem and verapamil) also interact with dronedarone. Because these CV drugs are frequently used in patients being treated for AF, clinicians are advised to be aware of these potential interactions and to closely monitor patients to adjust the doses to help prevent bradycardia or potential toxicity as appropriate.
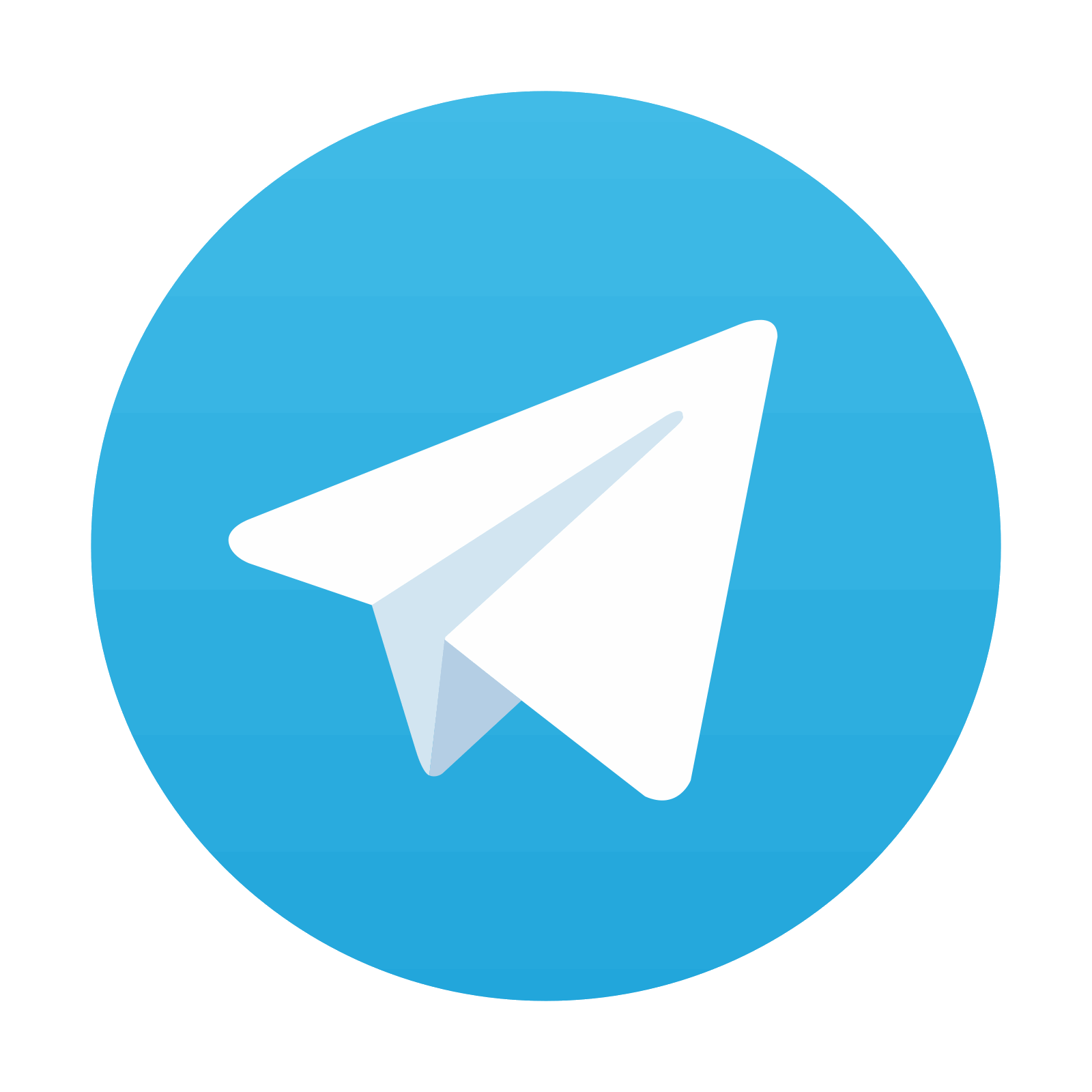
Stay updated, free articles. Join our Telegram channel
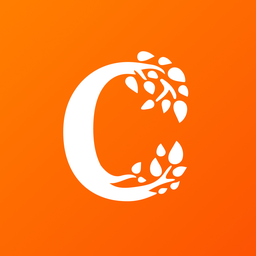
Full access? Get Clinical Tree
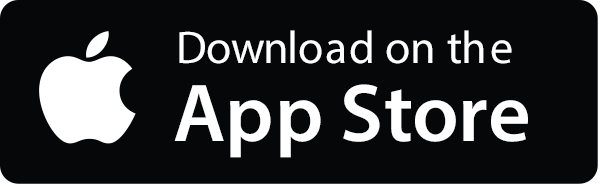
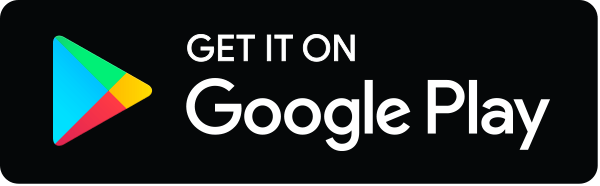