Abstract
A brief account of early embryo formation is followed by an overview of the salient features of heart development. Following this there is a detailed treatment of the processes involved in the construction of the functioning embryonic heart, including a focus on the genes involved. Short sections are devoted to pericardium, coronary arteries and conduction system, the systemic veins and the systemic arteries. Finally, the fetal circulation and its adaptation to post-natal life are described.
Human gene symbols are italicised, with all letters in uppercase (e.g. SHH for sonic hedgehog). Proteins have the same designation, but are not italicised, with all letters in uppercase (SHH). The same conventions are applied to chicken genes.
Rodent gene symbols are italicised, with only the first letter in uppercase and the remaining letters in lowercase (Shh). Protein designations are the same as the gene symbol but are not italicised and all are upper case (SHH).
Zebrafish gene symbols are italicised, with all letters in lowercase (shh). Protein designations are the same as the gene symbol but are not italicised; the first letter is in uppercase and the remaining letters are in lowercase (Shh) [1].
3.1 Introduction
The technical and ethical difficulties inherent in the study of early human embryos have determined that much of the detail of the early development of the heart is based on studies in mouse and chick embryos [2]. With minor differences in venous anatomy, mouse heart development is substantially the same as in the human [3]. This large volume of animal embryo experiments has been supplemented by the morphological examination of early human embryos. This examination, historically, has entailed reconstruction of serial histological sections of early embryos, but use of techniques such as magnetic resonance imaging (MRI) and Episcopic Fluorescence Image Capture more recently has yielded much more detailed information [4].
Although the heart is an organ largely derived from mesoderm (with small contributions from ectodermally derived neural crest), it does not develop in isolation. It is intimately related to its neighbouring structures such as the pharynx (Figure 3.1). Heart development is both influenced by and influences these surrounding structures. It is therefore no surprise that defects in the heart may frequently be associated with defects in these adjacent structures. From its earliest stages the heart is a beating structure containing flowing liquid, and hence mechanical forces are also important in shaping its development [5].
Figure 3.1 Relation of the developing heart to surrounding structures. A saggital section of a mouse embryo at approximately 12.5 days, equivalent to a human embryonic age of approximately 40 days. The heart within the pericardial cavity is present in the centre of the field. Dorsal to it is the trachea with the pharynx visible dorsal to this. Dorsal to this again and not in the field is the spine and notochord. The pharynx lies cephalad and the liver caudad.
3.2 Brief Recap of Relevant Early Human Embryonic Development
In the second week (days 7–14) post-fertilisation, the human embryo consists of a cellular, two-layered disc, one cell layer facing the amniotic cavity called the epiblast, and the other (facing the cavity of the yolk sac) called the hypoblast. During the third week the cells of the epiblast under the influence of BMP, FGF and WNT proliferate, migrate to the midline of the embryonic disc, where they are visible as the primitive streak, and there descend between the two cell layers and insinuate between them (a process known as gastrulation). The cells of the epiblast give rise to all three embryonic germ layers: those cells remaining in the epiblast constitute the embryonic ectoderm, other cells from the epiblast replace the cells of the hypoblast to form embryonic endoderm, and the insinuated cells between the ectoderm and endoderm constitute the embryonic mesoderm. Some of the mesenchymal cells coalesce in the midline to form the notochord, the structure that co-ordinates development of the axial nervous system and associated musculoskeletal structures. At the cranial end of the notochord is the prechordal plate where the endoderm and ectoderm are in contact (without interposed mesoderm) and which marks the site of the future mouth. The mesoderm on either side of the notochord is designated (with increasing distance from the midline) paraxial mesoderm, intermediate mesoderm and lateral mesoderm, the last extending to the edges of the embryonic disc. The paraxial mesoderm gives rise to the somites, which in turn give rise to the axial skeleton and its musculature. Spaces develop in the lateral mesoderm that gradually coalesce so that a cavity forms. The cavity will go on to form the pleural, pericardial and peritoneal cavities. The cavity thus formed divides the lateral mesoderm into two: the splanchnic (adjacent to the endoderm) and parietal or somatic (adjacent to the ectoderm) layers. The somatic mesoderm together with its associated ectoderm will form the embryonic body wall, whereas the splanchnic mesoderm will form the heart and intestine.
Some mesenchymal cells that have entered the mesoderm from the primitive streak migrate cranially on each side of the notochordal process cranial to the prechordal plate. They fuse cranially to form the cardiogenic mesoderm of the primary heart field (cardiac crescent) and are segregated into the splanchnic layer of lateral plate mesoderm [6]. The notochord induces the overlying ectoderm to form the neural tube by a process of invagination. As the neural tube closes, some cells at its margins detach and lie between the neural tube and the overlying reconstituted ectoderm as the neural crest. A subpopulation of these cells contributes to cardiac development. During the fourth week the embryo folds, converting the disc to a complex three-dimensional structure. The main driver of this folding is differential growth of the parts of the disc, bringing the caudal cranial and lateral edges together in the ventral midline where the opposite sides fuse.
3.3 Brief Summary of Heart Development
Within the cardiac crescent paired endothelium-lined tubes develop with their long axis in the long axis of the embryonic disc. These endothelium-lined tubes fuse in the midline to form a primitive heart tube. The inflow is caudal, a continuation of the primitive veins, and the outflow cranial and connected to the paired aortic arches. The surrounding mesoderm of the cardiac crescent differentiates to provide an investing sleeve of myocardium, both layers separated by extracellular matrix termed cardiac jelly. The pericardial cavity is present initially on the ventral surface, the heart tube being connected dorsally to the mesenchyme by the dorsal mesocardium. The primitive myocardium of this primary heart tube shows regular contractions by the third week. This heart tube elongates by addition of mesodermal cells at its two poles (and from the dorsal mesocardium until that structure involutes) and undergoes rightward looping. The atrial and ventricular chambers form by ballooning of the myocardium of the heart tube. Heart formation is completed with the development of valves, the conduction system and the formation of the coronary arteries by the ingrowth of extracardiac tissues derived from the neural crest, and from the pro-epicardium situated in the septum transversum [7]. We will now review this process in considerably more detail. Table 3.1 lists many of the major genes involved in heart development.
Gene | Location | Function | Defect |
---|---|---|---|
EOMES | 3p24.1 | The gene belongs to the TBR1 (T-box brain protein 1) subfamily of T-box genes sharing the common DNA-binding T-box domain. It is the earliest known specific gene for cardiomyocyte differentiation expressed during gastrulation. The gene encodes a protein transcription factor crucial for embryonic development of mesoderm and nervous system | Lack of the gene prevents development of the heart and is fatal |
BMP | BMP1: 8p21.3 BMP2: 20p12.3 | Drive myocardial specification and differentiation and negatively regulate FGF signalling. Bind and activate type 1 and 2 BMP receptors that result in phosphorylation of Smad1/5/8 proteins. Bmp signalling is inhibited by Smad 5 and 7 | BMP1 mutation: osteogenesis imperfecta type XIII BMP2 mutation: short stature, facial dysmorphism, skeletal abnormalities and cardiac defects |
MESP1 | 15q26.1 | Mesoderm Posterior bHLH Transcription factor 1. Required for primitive mesodermal cells to leave the primitive streak and participate in formation of early heart tube | Early embryonic death Cardia bifida |
ISL1 | 5q11.1 | Regulates signalling pathways required to coordinate second heart field deployment and is essential for heart tube elongation | |
GATA4 | 8p23.1 | Also required for testicular development | ASD-VSD, VSD ToF |
MEF2C | 5q14.3 | MEF2C belongs to the myocyte enhancer factor-2 (MEF2) family of transcription factors. MEF2C plays a pivotal role in myogenesis, development of the anterior heart field, neural crest and craniofacial development, and neurogenesis | AD mental retardation |
NKX2–5 | 5q35.1 | Human equivalent of Drosphila tinman gene | Holt–Oram syndrome ASD with conduction defects ToF VSD |
CX40 (GJA5) | 1q21.2 | Polymerise to form gap junctions for intercellular communication | Familial atrial fibrillation |
CX43 (GJA1) | 6q.22.31 | Polymerise to form gap junctions for intercellular communication | HLH AVSD |
NPPA (ANF) | 1p36.22 | Expression of ANF is one of the first markers of chamber formation | Atrial fibrillation |
TBX2 | 17q23.2 | Represses the chamber genetic programme and promotes cushion development in the AV canal and outflow tract | Mental retardation, multiple cardiac defects |
TBX3 | 12q24.21 | Represses the chamber genetic programme and promotes cushion development in the AV canal and outflow tract | |
TBX5 | 12q24.21 | Promotes chamber myocardial development | Holt–Oram syndrome |
TBX20 | 7p14.2 | Promotes chamber myocardial development | Overexpression in ToF |
FOXH1 | 8q24.3 | FOXH1 protein binds SMAD2 and activates an activin response element via binding the DNA motif TGT(G/T)(T/G)ATT | TGA Multiple VSD |
NOTCH | 9q34.3 | Local cell–cell signalling mechanism required for cardiac specification, progenitor cell differentiation, valve primordium formation and morphogenesis, ventricular trabeculation and compaction, and coronary vessel development | Aortic valve disease Alagille syndrome |
WNT Canonical | Binding of ligand stabilises intracellular β-catenin, which translocates to the nucleus, binds TCF/LEF and activates transcription. Overexpression of Wnt3a and Wnt 8 causes cardiomyocyte inhibition | ||
WNT Non-canonical | Non-canonical WNT signalling is mediated by intracellular calcium ions | ||
FGF8 | 10q24.32 | Required during looping by positively regulating differentiation | Hypogonadism |
FGF10 | 5p12 | Required during looping by positively regulating differentiation | |
FGFR1 | 8p11.23 | Required during looping by positively regulating differentiation | Skeletal dysplasia Hypogonadism |
TGFβ1 | 19q13.2 | Encodes TGFB, a multi-functional peptide that controls proliferation and differentiation in multiple cell types |
ASD = atrial septal defect; VSD = ventricular septal defect; ToF = tetralogy of Fallot; AD = autosomal dominant; HLH = hypoplastic left heart; AVSD = atrioventricular septal defect; TGA = transposition of the great arteries.
3.4 Early Development
Traditionally and of necessity, embryology of the heart has concentrated on morphogenesis and its coordination, on growth and on cellular differentiation. However, there is a series of steps that occurs before these, and on which these later processes are critically dependent, and that is not visible to the morphologist. These steps include the specification of precursor cells, their migration to the organ-forming region and the specification of cell types within the developing organ.
3.4.1 The Heart Fields
The term” heart field” describes a collection of mesodermal cells that contribute to the development of the heart. Two such fields are described, usually termed primary and secondary. The nomenclature is confusing and not all authors use the same terms for each. The fields change in position and with time [8]. In general, the primary heart field provides cells that form the primitive heart tube. The tube grows by addition of cells at its poles that are derived from the second heart field [9]. The first and second heart fields are temporally sequential [8]. The populations contributing to the heart are plastic, in that cells taken from a different location or developmental time point could contribute to the heart when placed in the appropriate location. This indicates that cellular environment is more important than the initial identity of the cells.
The cells that will form the heart fields are initially located in two small areas, one on either side of the midline in the epiblast of the bilaminar embryonic disc, close to the cranial part of the primitive streak. The earliest known committed cardiac precursors express the T-box transcription factor Eomesodermin\Tbr2 (EOMES) [10]. EOMES activates another transcription factor MESP1.
These progenitor cells migrate together through the primitive streak and form two plates of lateral mesoderm cells positioned anteriorly. The general specification of a heart field (cardiogenic mesoderm) has already started during this cellular migration. The progenitor cells migrate such that the medial–lateral arrangement of these cells will become the cranial–caudal axis of a linear heart tube. With the formation of the embryonic coelom, they segregate to the splanchnic mesoderm. The cells of this cardiac mesoderm express the cardiac-specific genes NKX2.5 and GATA-4 [11]. They become specified for heart development by interaction with surrounding structures. The adjacent foregut endoderm is thought to provide inductive signals to begin myocardial differentiation [12] (Figure 3.2). The foregut endoderm also plays a mechanical role in assembly of the heart tube. The endoderm around the oropharyngeal membrane actively contracts and pulls the bilateral fields of cardiogenic mesoderm towards the midline, permitting them to form the fused heart tube [13]. The primitive heart tube initially functions not so much to support the embryonic circulation as to provide a scaffold into which the cells from the secondary heart field migrate prior to chamber morphogenesis. Secondary heart field cells are first located medial to the cardiac crescent, and subsequently reside in mesoderm underlying the pharynx before they are added to the heart. The contribution of this population of cardiac progenitors to the heart was demonstrated by studies of the LIM transcription factor Islet1 (Isl1) that is a marker of the second heart field [14], although its expression is much broader than just the secondary heart field. The cranial part of the secondary heart field – the anterior heart field – which is marked by Fgf10 expression [15] and a specific enhancer of the Mef2c gene [16], contributes to the formation of right ventricular and outflow tract myocardium [17, 18], whereas cells in the posterior second heart field [14] expressing Isl1, but not anterior heart field markers, contribute to atrial myocardium [19]; that is to say, they are added at the venous pole of the developing heart. Myocardial progenitors from the left or right sides of the posterior secondary heart field contribute to the corresponding side of the common atrium, indicating that most cells do not migrate or mix either within the forming heart or across the secondary heart field [19]. The secondary heart field is patterned along the anterior–posterior axis of the mouse embryo; however, a detailed understanding of the molecular regulatory pathways governing this process is lacking [20].
3.4.2 The Heart Tube
Within the specified cardiac mesoderm anterior to the prechordal plate a primitive heart tube forms. The process begins when a plexus of endothelium-lined channels forms within the mesenchyme. These channels fuse to form bilateral endothelial tubes (Figure 3.3). Folding of the embryo brings these paired tubes together in the midline where they fuse to form a single endothelium-lined tube with a surrounding cuff of mesenchyme separated from it by extracellular matrix termed cardiac jelly. The unfused, paired inflow is caudal and the unfused, paired outflow is cranial. The tube is attached to the posterior body wall by dorsal mesocardium, while anteriorly it is surrounded by a cavity of the pericardium. Growth of the tube is by addition of mesodermal cells from the mesoderm of the inflow and outflow regions, and (until it breaks down) also the dorsal mesocardium.
Figure 3.3 Schematic drawing of a cross section of a trilaminar embryo before folding. The coelom is developing within the mesoderm, localising the paired heart tubes to the splanchnic mesoderm on the ventral aspect of the embryo. As the embryo folds, the lateral edges are brought into apposition, and the endoderm fuses to form the gut. The paired heart tubes are brought side by side and fuse in the midline ventral to the gut. The coelom forms the pericardial cavity. The ectoderm enfolds the other structures, and the amniotic cavity extends completely to surround the embryo.
By folding of the embryo, the lateral parts of the cardiac mesoderm are brought together, forming the ventral part of the heart tube. The inner curvature of the cardiac crescent forms the dorsal side of the tube, and is contiguous with the dorsal mesocardium, the attachment of the heart to the body wall. The peripheral part of the cardiac crescent will eventually face the transverse septum and forms the venous pole of the heart, whereas the central part of the crescent, which forms the outflow tract, is contiguous with the pharyngeal mesenchyme [21].
The cardiac precursors are initially situated anterior (cranial) and lateral to the future mouth, but posterior (caudal) to the mesoderm that forms the transverse septum, which contributes to the formation of the diaphragm. During the process of folding the cardiac precursors end up between the mouth at the cranial side, the diaphragm at the caudal side and ventral to the foregut, as in the adult situation (Figure 3.4) [9]. This intimate association of the cardiac and facial region during development is a possible explanation for the high incidence of combined cardiac and facial malformations [9]. The growth of the linear heart is not by division of in situ myocytes, but by addition from without. When being added to the heart, the fate of these cellular additions is not fixed. Their identity depends on their eventual location. The endocardium develops simultaneously with the myocardium and as a specialised endothelial cell type derived from the splanchnic mesoderm that via an unknown mechanism differs from the myocardial precursors [9].
(A) A representation of a saggital section through the unfolded embryo at the beginning of the fourth week. The cardiogenic mesoderm lies cephalad to the oropharyngeal membrane with the septum transversum lying further cephalad again.
(B) The box in A is expanded to show the detail. Note that the pericardial cavity at this stage is dorsal to the heart tube. Note also the close proximity of the endoderm of the foregut.
(C) With the marked expansion of the neural plate the heart moves ventrally and caudad.
(D) With further growth of the neural tissue the amniotic cavity extends further ventrally and caudally, and the developing heart now comes to lie caudad to the oropharyngeal membrane with the pericardial cavity ventrally. With lateral folding of the embryo the foregut develops, and the stalk of the yolk sac is constricted. The septum transversum in which the liver will develop now lies ventral and caudad to the heart.
Figure 3.4 A diagrammatic representation of the shift in position of the heart relative to the gut and liver.
3.4.3 Contraction
The myocardium shows regular contractions by the third week. This indicates that the functional adaptations required for contraction, namely contractile proteins, sarcoplasmic reticulum and gap junctions, are already present in the myocardial cells of the primitive heart tube. It would appear that the initial contractions, at least in the chick embryo, are not essential for tissue oxygen and nutrient supply, but have more to do with mechanical stimuli for further development of the heart [5]. Early blood flow in the embryo is dependent on the yolk sac and the development of the vitelline circulation. When the placental circulation becomes functional, both extraembryonic circulations send blood to the developing heart. Haemodynamic changes in the extraembryonic vitelline or placental circulations can alter normal heart development to induce cardiac abnormalities [22].
3.5 Looping of the Heart Tube
Looping of the straight heart tube begins at around day 22 following activation of a gene cascade that determines right–left symmetry. These genes are already expressed in the cardiac mesoderm before formation of the heart tube and include the genes LEFTY, NODAL, and PITX2, of which PITX2 is the controlling gene [23, 24]. Looping is caused by elongation of the tube, fixed at its caudal and cranial ends, within the confines of the pericardial cavity [25]. This elongation results from addition of cells at each end from the secondary heart field, and by dissolution of the tethering dorsal mesocardium. During the early phase of looping the straight heart tube bends towards the ventral body wall and undergoes torsion around its central axis such that it acquires the configuration of a helix with a single counter-clockwise (left-handed) twist. This loop looks like the letter “C” when viewed in a frontal two-dimensional projection with the convexity of the “C” pointing to the right side of the embryo. The heart loop then acquires a more complex helical shape, which resembles the letter “S” as viewed in a frontal two-dimensional projection. The configuration of the “S-shaped” heart loop has been termed a “helical perversion” [26]. A helical perversion connects two helical segments of opposite handedness within the same helically coiled object (a two-handed helix). Applied to the embryonic heart, the two-handed helix consists of a caudal limb with a left-handed twist and a cranial limb with a right-handed twist. These two are connected by a curved loop convex to the right (Figure 3.5). Following the direction of blood flow, this heart loop configuration may be termed a “left–right-handed helix”.
(A) A schematic drawing of the looping heart tube at approximately 26 days. The anterior wall of the pericardium has been cut away. The anterior part of the neural tube is visible above its superior aspect. At the inferior part of the field the two sinus horns fuse with the atrium. The heart tube then loops anticlockwise to the right. At the midline it then loops clockwise to result in an “S”-shaped tube. It exits the pericardium superiorly to enter the aortic sac. The two coils at the edge of the diagram illustrate the direction of looping.
(B) A simplified model of the looping heart viewed from anteriorly and slightly to the left. The sinus horns are inferior and posterior and the aortic sac and dorsal aorta superior and anterior. The deep scores in the tube superiorly and inferiorly represent the approximate sites of the pericardial attachment.
Figure 3.5 Looping of the heart.
The direction of looping is not random but is controlled by genes not yet understood and not under the control of PITX2. It has been shown in a mechanical model that looping will occur to the right if the venous pole is moved to the left of the midline [27]. The looping causes the segments of the tube to be brought into close proximity on the inner curvature of the loop. This is absolutely essential for establishing the correct connections of the chambers. Actin polymerisation-driven myocardial cell shape changes have been found to contribute to the bending of the heart tube [28, 29]. The looped heart tube has unidirectional blood flow. This flow was originally thought to be peristaltic but is claimed to be more akin to a Liebau pump. Liebau pumping is the unidirectional flow obtained when a more or less elastic tube containing a fluid is periodically squeezed. It is pulsatile [30]. The developing atrioventricular cushions function as primitive valves to permit unidirectional flow of blood in the looped heart tube [31].
3.6 Development of the Chambers and Septation
Septation of the ventricles occurs between 5 and 7.5 weeks of gestation. The atria and ventricles develop by ballooning growth from the heart tube, the atria from the dorsal aspect and the ventricles from the ventral aspect (Figure 3.6) [9]. In the atrial segment this growth is bilateral and in parallel, whereas in the ventricular segments it is unilateral and in sequence. Thus, in the atria it is possible to develop isomerism – it is impossible for this to occur in the ventricles. The ballooning myocardium shows trabeculations and does not have associated cardiac jelly between it and the endocardium with the result that histologically, the cardiac chambers are characterised by trabeculation and absence of cardiac jelly [9] (Figure 3.7). By this differential growth the cardiac jelly becomes confined to the atrioventricular junctions and outflow tracts (Figure 3.8). The endocardial cells of the atrioventricular canal and outflow tracts give rise to cells that populate the underlying cardiac jelly to form cardiac cushions that contribute to the septation of these regions. At the same time, the atria and ventricles develop myocardial trabeculations. The myocardium that forms the chambers shows specific gene expression (Anf, Cx40, Cx43) but does not show Tbx 2 and Tbx3 expression, which are repressors of the process and persist in the primitive myocardium of the primary heart tube [32]. Interestingly, the early markers of chamber formation Anf and Cx40 remain restricted to the original trabeculated myocardium, whereas the compact ventricular layer does not express these markers [33]. The initially formed atrial chamber myocardium only gives rise to the trabeculated, atrial appendages in the formed heart. All smooth-walled myocardium found in the fully grown heart is added later during development. How the left and right ventricles obtain their different morphology is not understood, albeit some differences between the left and the right ventricle in terms of gene expression are known. For instance, the cardiac transcription factor Tbx5 is expressed in a gradient tapering off towards the right ventricle [9]. Ventricular myocardial compaction is, thus, not a process by which trabeculated myocardium becomes compact, but primarily a process by which the myocardium at the epicardial side of the ventricular wall proliferates to form the compact layer. When the compact outer layer starts to form, proliferation in the ventricular trabeculations ceases [9].
Figure 3.6 Ballooning of atria and ventricles. A schematic model of the heart at approximately 38 days. The outflow tract is to the left and the sinus venosus at the posterior aspect of the right atrium. The blue area outlines the original heart tube from the sinus venosus through the atrioventricular canal to the outflow. The atria balloon out from the tube, one on either side, but the ventricles balloon out in sequence, the left ventricle on the right of the picture and the right ventricle on the left of the picture. The supraventricular foramen is visible above the crest of the muscular interventricular septum. It is obvious that the interventricular septum is formed of the apposed walls of the ballooning right and left ventricles and represents an “outgrowth” of the heart rather than an “ingrowth” into the ventricles.
Figure 3.7 Ventricular trabeculation. A section from the ventricular apex of a mouse embryo at the same age as that above. The trabeculae contain myocytes and are covered with endocardial cells. At the pericardial surface there is a continuous layer of mesothelial cells and the underlying myocardium is compacted. Mitotic figures are visible in the compacted layer of myocardium. The compacted layer forms by addition of cells to the outer aspect of the myocardium rather than fusion (compaction) of the trabeculae.
Figure 3.8 Endocardial cushions. A section through the thorax of a mouse embryo at age 13.5 days, equivalent to a human embryo at 44 days. One of the atrioventricular valves and the aortic valve are seen and contain myxoid cellular tissue derived from the cardiac jelly. Elsewhere in the myocardium, the cardiac jelly is lost.
There is a gradient of strain across the ventricular wall, greatest on the inner surface and least on the outer surface. There is a corresponding increase in myocyte proliferation in the outer compact layer of the myocardium than in the inner trabecular component [34].
The curvature of the heart depends on cell shape changes at the cellular level caused by a complex interplay of haemodynamic shear stress, contractive wall strain and electrical activity. NOTCH, ERBB and Ephrin play a role in trabeculation, whereas NOTCH, BMP and FGF play a role in compact myocardium development [35].
Initially, because the atrial segment is connected to the left ventricular segment, there is no direct connection with the right ventricle, but blood can flow from the atria to the right ventricle via the interventricular foramen (Figure 3.9). The atrial–right ventricular connection is established by growth of the ventricular inlet to the right. Likewise, the outlet is connected initially solely to the right ventricle, but by a similar differential growth, the outlet comes to overlie both ventricles. The ventricular septum grows from the apex of the heart loop between the left and right ventricular segments.
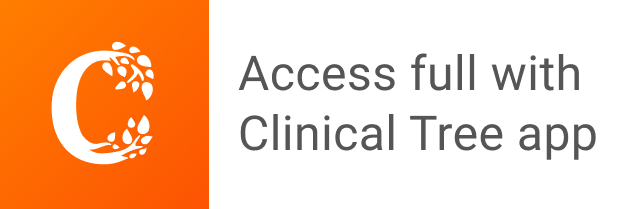