(Fig. 10.1). Elimination occurs from the central compartment and can be described by the elimination rate constant. By measuring plasma concentrations over a time period from the injection of the drug, it is possible to describe the concentration-versus-time profile for the drug (Fig. 10.2) (22). Distribution and elimination phases can be determined and a mathematical description (model) of the change in drug concentration versus time can be developed.
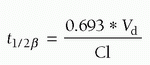
as drug administration continues, there is accumulation of drug in tissues over time, which increases with the duration of drug infusion. On termination of the infusion, offset of drug effect then depends on redistribution of the drug out of tissues (greater with longer infusions) back into the central compartment as well as the rate of elimination. Thus, when a drug has accumulated in peripheral tissues, reliance on the elimination half-time will not adequately predict termination of drug effect because it does not take into account the role of redistribution. This has led to the introduction of the term context-sensitive half-time as a better description of the phenomenon of increased duration of drug effect with increased drug infusion time (Fig. 10.3) (44).
TABLE 10.1. Definition of basic pharmacokinetic parameters | |||||||||||||||||||||||||||||||||||||||
---|---|---|---|---|---|---|---|---|---|---|---|---|---|---|---|---|---|---|---|---|---|---|---|---|---|---|---|---|---|---|---|---|---|---|---|---|---|---|---|
|
of the population studied (e.g., young, healthy men versus elderly women with congestive heart failure [CHF]). Drug in the blood exists in several forms, including that which is free (active), bound to plasma proteins (e.g., albumin, and therefore subject to changes in plasma protein concentrations), or sequestered in red blood cells. CPB has the potential to alter all of these factors, which makes the description of pharmacokinetic parameters during CPB problematic.

TABLE 10.2. Examples of G-protein-coupled receptors and the intracellular second messengers they generate | ||||||||||||||||||||||||||||||
---|---|---|---|---|---|---|---|---|---|---|---|---|---|---|---|---|---|---|---|---|---|---|---|---|---|---|---|---|---|---|
|
regulation of several different Ca2+-specific channels in the plasma membrane and by its release from intracellular storage sites (Fig. 10.12) (69,86,89). Ca2+-dependent ion channels are opened by electrical depolarization, phosphorylation by a cAMP-dependent protein kinase, and by Gs, K+, and Ca2+ itself (89). Opening of the channel may be inhibited by other proteins (e.g., Gi).
An immediate reduction in concentrations of circulating proteins such as albumin and a1-acid glycoprotein (α1AGP). This has implications for protein binding of drugs resulting from alteration in the ratio of bound drug to free drug in the circulation (5,6,7,8,9,10,95,96,97,98,99,100,101,102,103).
An immediate reduction in red blood cell concentration, which has implications for compounds that are sequestered to a significant degree in red blood cells (3,8,104,105).
An immediate reduction in the amount of free drug in the circulation at the initiation of CPB. This will reduce the amount of drug available for interaction with the receptor, with the potential for adverse events, for example, lightening of the level of anesthesia (5,6,7,36,106).
Alteration in organ blood flow, which may affect drug distribution and clearance (107).
lipid solubility relative to the volume of the CPB prime. Thus, following intravenous administration, the tissues serve as a reservoir that sequesters the drug. At the onset of CPB, when plasma concentrations fall due to hemodilution, the drug moves down its concentration gradient from the tissue stores to plasma. As protein concentrations fall, the free drug concentration increases to reestablish an equilibrium based on its solubility in plasma (8,11,101). Because free drug concentration is responsible for drug effect (109,110), the increase in free drug concentration from altered protein binding may explain the increased pharmacodynamic effect observed during and after CPB (9,10,111).
Peripheral vasoconstriction may decrease absorption of drugs administered other than by the intravenous route (119).
Fluid extravasation may alter drug distribution from central to peripheral compartments (i.e., changes in the volume of distribution, Vd) (94,120).
Vasoconstriction may reduce the rate of reuptake of drug from peripheral tissues to the central compartment (120,121).
Temperature-induced reductions in enzyme-mediated biotransformation may decrease clearance of drugs and increase their elimination half-time (Fig. 10.16) (120,121,122,123,124,125,126,127,128,129,130,131).
Changes in organ perfusion may produce altered renal drug excretion as a result of decreased renal perfusion, glomerular filtration rate, and tubular secretion (132). However, studies comparing renal function during normothermic versus hypothermic bypass have not demonstrated any clinically important differences (117,130,133,134).
Hypothermia-induced increases in drug solubility in blood of volatile anesthetics (135).
esmolol, remifentanil, clevidipine, atracurium, and cis-atracurium) (123,124,125,127). The net result is a prolonged drug effect that requires dosage reductions.
in whom postoperative lung concentrations of levofloxacin administered in the intensive care unit (ICU) were lower among those undergoing CPB versus a group not undergoing CPB. This finding was attributed to a higher degree of atelectasis (and hence altered tissue distribution) in postoperative CPB patients (151).
PLP group was associated with an increase in blood pressure and Bispectral Index (BIS) scores (BIS—a measure of anesthetic level), indicative of lightening of anesthesia, a finding which was not observed in the PMP group.
theory the use of a cell saver device might have an effect on clearance of protein-bound drugs, this has not been demonstrated to be a clinical concern to date (197).
TABLE 10.3. Factors influencing movement across a membrane | ||||||||||||
---|---|---|---|---|---|---|---|---|---|---|---|---|
|
slower return of drug from the effect site to the central compartment (a pharmacokinetic difference) (216). In contrast, a true difference in CNS sensitivity to opioids (fentanyl, alfentanil, and remifentanil) (217,218) and volatile agents (219) has been described in the elderly. For the elderly patient undergoing CPB, a reduction in dose to limit the high free drug concentrations and the potential for prolonged or toxic drug effects therefore seems prudent.
TABLE 10.4. Muscle relaxants and hypothermic cardiopulmonary bypass | |||||||||||||||||||||||||||||||||||||||||||||||||||||||||||||||||||||||||||
---|---|---|---|---|---|---|---|---|---|---|---|---|---|---|---|---|---|---|---|---|---|---|---|---|---|---|---|---|---|---|---|---|---|---|---|---|---|---|---|---|---|---|---|---|---|---|---|---|---|---|---|---|---|---|---|---|---|---|---|---|---|---|---|---|---|---|---|---|---|---|---|---|---|---|---|
|
of excitatory amino acid receptors in the spinal cord triggers intracellular events leading to Ca2+ release and phosphokinase C (PKC) activation. PKC is associated with a number of neuronal changes, including development of hyperalgesia (290). As tolerance to morphine administration shares many of the same pathways (290), studies in animals and humans have also implicated these pathways in the development of hyperalgesia (so-called opioid-induced hyperalgesia [OIH]) (291,293). Whether hyperalgesia develops in the perioperative period is debated, but recent meta-analysis concluded that, at least for the administration of remifentanil, it does (294). Representative studies, in patients undergoing cardiac surgery, include that of Richebe and colleagues (295) who examined the incidence of hyperalgesia following remifentanil administration by CACI with a target concentration of 7 ng/mL as compared to a group of patients receiving a zero-order infusion of 0.3 µg/kg/min. Use of the targeted infusion led to a reduction in total remifentanil dose and a reduction in hyperalgesia. In a randomized study of 40 patients undergoing coronary artery bypass graft (CABG) surgery comparing sufentanil targeted to a plasma concentration of 0.4 or 0.8 ng/mL, it was observed that the higher-dose sufentanil group had increased postoperative analgesic requirements, but assessments for hyperalgesia indicated that, while present and diminishing over time, the degree of hyperalgesia was not different between the two groups (296). In contrast, in a randomized study of 90 patients undergoing cardiac surgery and receiving either sufentanil 0.3 µg/kg/min intraoperatively or placebo (in addition to a sufentanil/propofol-based anesthetic), no difference in postoperative analgesic requirements was observed. Although the authors concluded that hyperalgesia did not occur (297), it must be acknowledged that it was not specifically tested for as in the studies of Fechner et al. (296) and Richebe et al. (295).
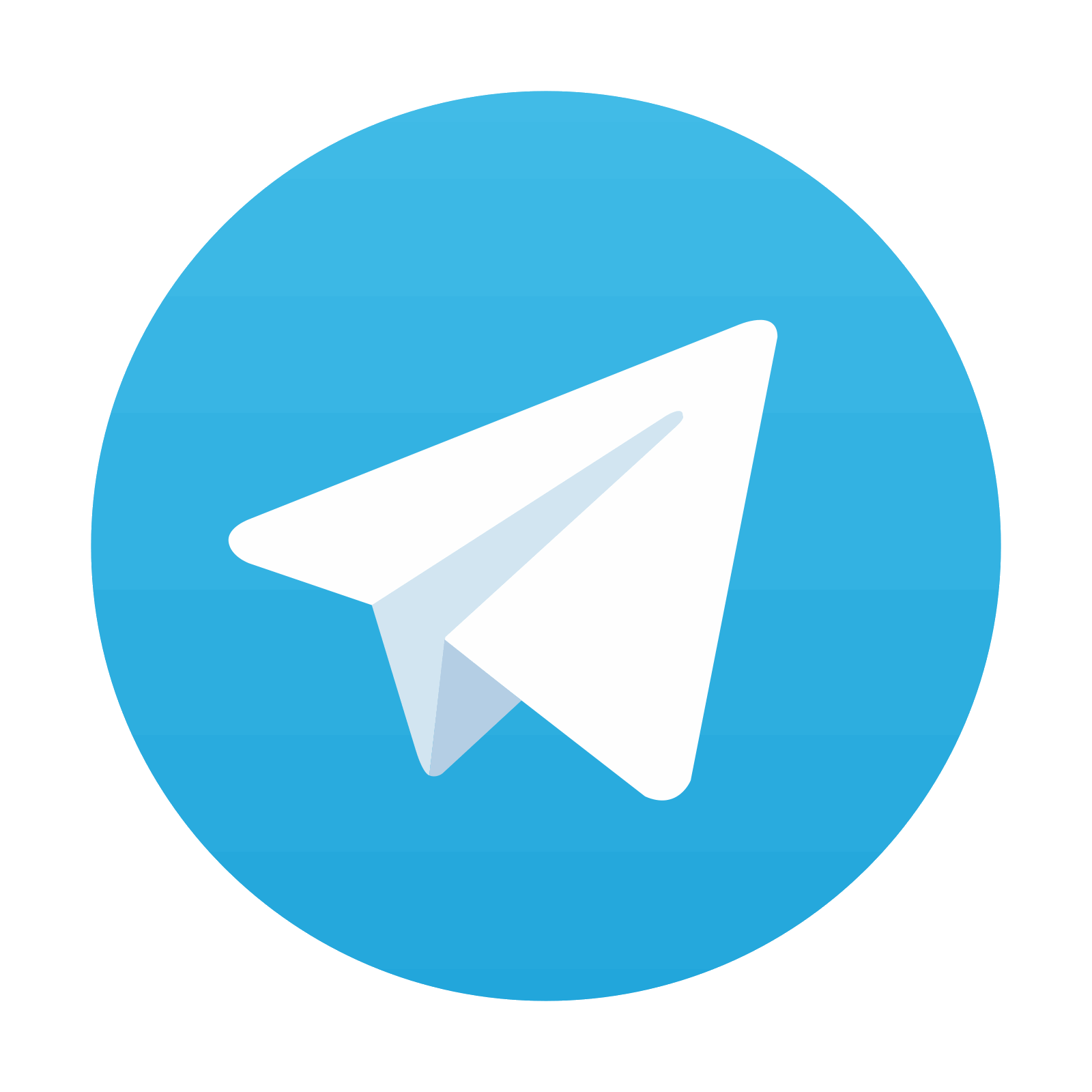
Stay updated, free articles. Join our Telegram channel
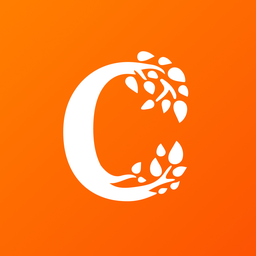
Full access? Get Clinical Tree
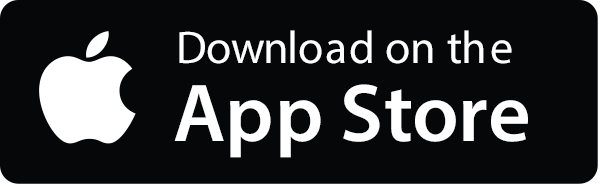
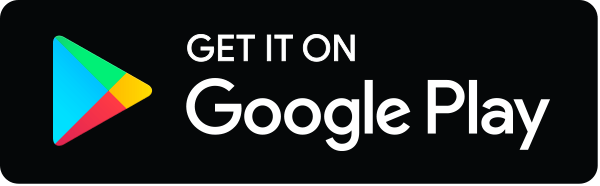