Cellular and Molecular Pathology of Lung Cancer | 3 |
INTRODUCTION
Traditionally, clinical treatment options for lung cancer were based on the distinction between small cell lung carcinoma (SCLC, 13% of cases) and non–small-cell lung carcinoma (NSCLC, 83% of cases) (1), the two major types of lung cancer. Although this distinction is still relevant, this basic categorization of tumor types is no longer considered sufficient. Pathologists are under greater pressure to precisely subclassify lung cancers, even when presented with very small tumors or cytologic samples, because histologic subtyping is increasingly important for making molecular testing decisions and for the selection of appropriate therapy (1). Unfortunately, most patients with lung cancer present with advanced-stage disease and the overall prognosis is poor, with an overall 5-year survival rate of only 15% (2). Major advances in understanding the molecular pathogenesis of lung cancer have led to new strategies for early detection, diagnosis, staging, and therapy. The personalized or precision medicine approach has increasingly become a day-to-day reality in the treatment of lung cancer, and having a clear understanding of the molecular alterations within tumors will be crucial to improving patient outcomes (3–5). Pathologists play a crucial role in facilitating clear and timely communication between the clinical oncology care team and the molecular diagnostic laboratory. This chapter provides a broad overview of several of the driver oncogenes that are important in the pathogenesis of NSCLC and have emerged as targets for therapeutic approaches.
HISTOLOGIC SUBTYPING OF LUNG CANCER
Clinical treatment options were traditionally based on the distinction between SCLC and NSCLC, without major therapeutic interest in further subclassification. However, the advent of molecular profiling and targeted therapy renewed interest in the distinguishing between the major subtypes of NSCLC: adenocarcinoma (ADC, 39%) (2), squamous cell carcinoma (SqCC; 20%), and large cell lung carcinoma (LCLC, 3%) (4,6,7) (Figure 3.1). LCLC occurs throughout the lung and usually grows and spreads at a rapid rate. Other subtypes, including sarcomatoid carcinoma and neuroendocrine large cell carcinoma, represent a very small proportion of all NSCLC cases.
Figure 3.1 Microphotographs of H&E Stained Sections of the Four Major Subtypes of Lung Cancer: (A) Squamous Cell Carcinoma, (B) Adenocarcinoma, (C) Large Cell Carcinoma, and (D) Small Cell Carcinoma, H&E, x200
Squamous Cell Carcinoma
SqCC generally occurs in the central portion of the lung and is highly associated with tobacco smoking. Classical SqCC is generally recognized under light microscopy by its distinctive areas of keratinization and an associated inflammatory component, especially in tumors that have undergone cavitation (Figure 3.1). Less differentiated forms of SqCC, with smaller cells and without keratinization, may resemble basal cell layers of squamous epithelium (7). In these cases, immunohistochemistry (IHC) may be required to detect the presence of specific types of cytokeratin and the absence of neuroendocrine markers (Table 3.1).
Adenocarcinoma
ADC is a relatively slow-growing lung cancer usually discovered in the periphery of the lung. It occurs most often in smokers, but it is the most common form of lung cancer found in nonsmokers (7). ADC includes a morphologically heterogeneous group of tumors characterized by gland formation (acinar pattern), papillary or micropapillary structures, or solid growth with mucin production (Figure 3.2) (7). Adenocarcinoma in situ (AIS), previously termed as bronchioloalveolar carcinoma, is defined as a small (≤3 cm) solitary tumor with a pure alveolar epithelial appearance, lepidic growth, and lack of invasion of the interstitium. AIS is further divided into mucinous and nonmucinous subtypes (Figure 3.2) (6). In clinical practice, however, the most common subtype is invasive ADC showing a combined pattern of growth, with conventional acinar or papillary ADC mixed with areas of AIS. Such tumors are classified as a mixed subtype ADC (6,7).
Figure 3.2 Microphotographs of H&E Stained Sections of the Common Subtypes of Lung Adenocarcinoma: (A) Acinar, (B) Papillary, (C) Micropapillary, (D) Solid, (E) Lepidic, Nonmucinous, and (F) Lepidic, Mucinous, H&E, x200
Small Cell Lung Cancer
SCLC usually arises in the bronchi in the central portion of the lung. It is an aggressive cancer that spreads to lymph nodes and distant sites early in its course and tends to grow much faster than NSCLC (8). The major histologic feature that distinguishes SCLC from NSCLC is cell size. Typically, the cells in SCLC are roughly twice the size of lymphocytes when subjectively assessed under light microscopy. Additional features that distinguish SCLC from NSCLC include hyperchromatic appearance, small amounts of cytoplasm (i.e., high nucleus-to-cytoplasm ratio), cohesive sheets of small “blue” cells, crush artifact, necrosis, cellular fragility, and a high mitotic rate with an average of 80 mitoses/2 mm2. When the classic features of SCLC are present, morphologic criteria alone are often diagnostic and support high interobserver reliability (8). Although IHC is useful in the diagnosis of SCLC, the most important stain is a good-quality hematoxylin and eosin (H&E) stain.
Immunohistochemistry
The most recent histologic classification of lung cancer published by the World Health Organization in 2015 incorporates the relevant genetic and IHC features of the different tumor subtypes (7). Major advances in lung cancer biology, diagnosis, and treatment have occurred in the past 10 years, and there is an even greater need for an integrated multidisciplinary approach to the diagnosis and management of NSCLC. Lung cancers are increasingly diagnosed and staged via transthoracic core needle biopsy or fine needle aspiration, endobronchial ultrasound (EBUS)-guided transbronchial needle aspiration, or endoscopic ultrasound (EUS)-guided fine needle aspiration (9). These techniques frequently result in small histologic or cytologic samples, which may be a limiting factor in distinguishing NSCLC subtypes and in obtaining an accurate histologic and molecular diagnosis.
Poorly differentiated ADC and SqCC can be indistinguishable by routine microscopy, particularly in small histologic and cytologic specimens. When presented with small samples, particularly when the tumor is poorly differentiated, we need to integrate morphology with IHC analysis to provide a precise diagnosis. In such situations, a useful IHC panel includes thyroid transcription factor (TTF1), the novel aspartic proteinases of the pepsin family (napsin A and B) and surfactants A and B, which are expressed in ADC, and p40, p63, and cytokeratin 5/6, which are expressed in SqCC (Figure 3.3 and Table 3.1) (7). In addition, staining for mucin is useful for the diagnosis of ADC.
Figure 3.3 Microphotographs of Immunohistochemical Analysis to Distinguish Lung Adenocarcinoma (ADC) From Squamous Cell Carcinoma (SqCC): (A) H&E of ADC, (B) H&E of SqCC, (C) Thyroid Transcription Factor (TTF1) Positive in ADC, (D) TTF1 Negative in SqCC, (E) p40 Negative in ADC, and (F) p40 Positive in SqCC. H&E, Hematoxylin, and Eosin, x200 and IHC, Immunohistochemistry, x200
Poorly differentiated metastases from distant sites may also need to be distinguished from primary NSCLC. Stains that are classically negative in NSCLC such as cytokeratin 20 (typically positive in colon cancer) and estrogen and progesterone receptors (typically positive in breast cancer) can help to distinguish the tissue of origin for an ADC found in the lung. SCLC can be positive for TTF1, but otherwise the staining pattern of SCLC should be distinct from that of NSCLC. As a neuroendocrine tumor, SCLC is typically positive for synaptophysin, CD56 neural cell adhesion molecule (NCAM), chromogranin, or neuron-specific enolase, whereas NSCLC is negative for these stains (Table 3.1) (7). While obtaining an accurate histologic diagnosis is important, it is also imperative to perform only essential IHC stains in order to ensure adequate tissue to maximize the yield for molecular testing.
MOLECULAR DIVERSITY IN NSCLC
At present, epidermal growth factor receptor (EGFR) mutation and anaplastic lymphoma kinase (ALK) rearrangement are the two best-characterized molecular alterations associated with targeted therapy in NSCLC, particularly ADC. However, the frequency of these two derangements combined is less than 20% in most patient populations. Thus, extensive efforts are ongoing to identify additional recurrent aberrations and to understand aspects of the biology of lung cancer that can be exploited for targeted therapy (Figure 3.4). On the basis of these efforts, other genomic alterations may become part of the routine analysis of NSCLC in the near future (5). Although the list of emerging genomic alterations is extensive, we present here those that may have clinical relevance as molecular targets in NSCLC (Table 3.2).
Epidermal Growth Factor Receptor
The EGFR gene is located on the short arm of chromosome 7 at position 12 (10). The protein encoded by this gene is a transmembrane glycoprotein that is a member of the protein kinase superfamily (10). EGFR is overexpressed in 40% to 80% of NSCLCs and many other epithelial cancers. Approximately 10% of patients with NSCLC in the United States and 35% in East Asia have tumor-associated EGFR mutations (11). The most common EGFR mutations, generally termed classic or sensitizing mutations, are in-frame deletions in exon 19 (around amino acid residues 747–750; 45% of EGFR mutations) and the L858R point mutation in exon 21 (40% of EGFR mutations) (12). The third most common type of EGFR mutation is in-frame insertions in exon 20 (5%–10%) (13). Other recurrent mutations include exon 18 point mutations at G719 (3%), exon 21 L861Q mutations (2%), and in-frame insertions in exon 19 (<1%) (12). EGFR mutations are more often found in tumors with ADC histology from female never-smokers (11,14). However, EGFR mutations can rarely be found in other lung cancer histologic subtypes in never-smokers. The paucity of classic EGFR mutations in SqCC of the lung has led to the widespread recommendation to obtain genotyping (usually complete or allele-specific sequencing of key regions of exons 18–21) only in non-squamous NSCLCs, except in cases with mixed histology or high clinical suspicion (e.g., never-smokers). The College of American Pathologists, the International Association for the Study of Lung Cancer, and the Association for Molecular Pathology recommend rapid testing for EGFR mutation and ALK rearrangement in all patients with advanced-stage ADC (15). The etiology of the initial genomic insult that either leads to or selects for EGFR mutations in lung tissue remains elusive. Interestingly, rare inherited germline EGFR mutations, such as T790M and V843I, are associated with increased familial clustering of EGFR-mutated NSCLC in which tumors develop when a somatic classic EGFR mutation associates with the inherited allele.
Figure 3.4 Relative Frequencies of Molecular Targets in Lung Adenocarcinoma (A) and Squamous Cell Carcinoma (B). The Number of Novel Molecular Targets Will Increase as Further Research Continues to Discover and Validate Them
Tumors with EGFR mutations are susceptible to treatment with EGFR tyrosine kinase inhibitors (EGFR-TKIs) such as gefitinib and erlotinib (16). However, relapse eventually occurs and resistance to 1st generation EGFR-TKIs is usually associated with a second mutation in exon 20 (T790M or D7961Y), MET amplification, or PI3KCA mutation (5). Interestingly, “transformation” to SCLC has also been described in a subset of ADC exhibiting resistance to EGFR-TKIs (5). Gene sequencing is the most comprehensive method of EGFR mutation testing. IHC for EGFR is not currently recommended because the expression of EGFR by IHC does not necessarily correlate with the presence or absence of an EGFR mutation or responsiveness to EGFR-directed therapy (15).
Anaplastic Lymphoma Kinase
ALK was originally discovered in chromosomal translocations leading to the production of fusion proteins consisting of the C-terminal kinase domain of ALK and the N-terminal portions of a variety of other genes (17). The ALK gene is located on the short arm of chromosome 2 at position 23 (17). Translocation of ALK has been identified in approximately 3% to 7% of NSCLCs in both white and Asian populations (18,19). Nucleophosmin (NPM1) is the most common fusion partner of ALK, primarily in hematologic malignancies, accounting for 80% of ALK translocations, but at least six other fusion partners have been identified (20).
In NSCLC, the most common ALK fusion partner is the echinoderm microtubule-associated protein-like 4 (EML4) gene. EML4-ALK is an aberrant fusion gene that encodes for a chimeric protein with constitutive kinase activity (21). EML4-ALK fusions are more commonly found in younger patients who have never smoked or have a history of light smoking (<10 pack years) (19,20), and in patients with ADC with acinar or signet ring cell histology (18,22). In lung SqCC, the estimated prevalence of ALK rearrangement is approximately 1%. Other fusion partners for ALK, such as KIF5B and TFG, have also been described in NSCLC (23). In the majority of cases, ALK rearrangements are mutually exclusive with other oncogenic mutations, such as those in EGFR and KRAS (18,19).
Clinical trials demonstrate that patients with ALK-rearranged NSCLC can be successfully treated with crizotinib, an ALK inhibitor, and that crizotinib is superior to chemotherapy in both the first- and second-line settings (24). In addition, next-generation ALK inhibitors, such as alectinib and ceritinib, have shown excellent efficacy for patients with ALK-rearranged NSCLC. However, ultimately acquired resistance develops to ALK inhibitors. Potential mechanisms of resistance to ALK inhibitors include secondary mutations or copy number gain of the ALK gene and activation of bypass pathways, including EGFR, KRAS, KIT, MET, and IGF-1R. Therapeutic strategies to overcome these resistance mechanisms have been proposed, including next-generation ALK inhibitors, agents that inhibit bypass pathways, and heat shock protein 90 inhibitors (25).
In clinical practice, fluorescence in situ hybridization (FISH) is the primary method used to identify gene copy number changes and structural variations due to ALK rearrangements. For NSCLC, FISH using the Vysis ALK Break Apart Probe Kit (Abbott Molecular) was approved as a companion diagnostic test along with crizotinib on the basis of the clinical responses seen in patients with ALK-rearranged tumors (26). The use of IHC for ALK protein detection is based on the premise that ALK protein is normally absent in the lung and therefore, the overexpression of ALK protein infers an underlying rearrangement of the ALK gene, leading to constitutive activation and subsequent overexpression of the protein (26). Although IHC is used as a prescreening method to detect ALK protein, confirmation by FISH is required because IHC is subject to technical issues such as tissue fixation, antibody clone used, endogenous peroxidase activity, necrosis/crush artifact, and interobserver variability.
ROS1
The ROS1 proto-oncogene is located on the long arm of chromosome 6 at position 22. It is a member of the insulin receptor gene family and is a type I integral membrane protein with tyrosine kinase activity, which may function as a growth or differentiation factor receptor. ROS1 rearrangements lead to constitutively active fusion proteins and are detected in approximately 1% to 2% of NSCLCs (27). ROS1 rearrangements are associated with ADC, are most commonly found in light or never-smokers and young patients (<50 years of age) (27), and are mutually exclusive with EGFR mutations, KRAS mutations, and ALK rearrangements (28). Several types of ROS1 rearrangements, such as SLC34A2-ROS1, CD74-ROS1, EZR-ROS1, TPM3-ROS1, and SDC4-ROS1, have been described in NSCLC (23,28).
In recent studies, patients with advanced NSCLC harboring an ROS1 rearrangement derived great benefit from treatment with crizotinib, which targets ROS1 in addition to ALK (27). For this reason, the U.S. Food and Drug Administration (FDA) recently expanded the use of crizotinib to patients with metastatic NSCLC whose tumors harbor an ROS1 rearrangement. Crizotinib is the first and only FDA-approved treatment for patients with ROS1-rearranged NSCLC.
RET
The RET gene is located on the long arm of chromosome 10 at position 11.2. It encodes for a tyrosine kinase that is involved in cellular proliferation, migration, and differentiation (29). Although RET point mutations and fusions have long been recognized in medullary and papillary thyroid carcinoma, respectively, RET rearrangements involving kinesin family member 5B (KIF5B) were only recently discovered in NSCLC (28,30). Alternative RET fusion partners, such as CCDC6-RET, NCOA4-RET, and TRIM33-RET, have since been described (31). In early studies, RET rearrangements were identified in approximately 1% to 2% of NSCLCs (28,32). RET fusions are associated with specific clinicopathologic features, such as smoking status, young age (≤60 years), and ADC histology, especially poorly differentiated tumors (31). RET rearrangements are largely mutually exclusive with genetic alterations in other oncogenic drivers, such as EGFR, KRAS, ALK, and ROS1 (28,31,32), suggesting that RET-rearrangement defines a new, distinct, molecular subset of NSCLC.
RET fusions are oncogenic, and in vitro studies show that small-molecule inhibitors, such as vandetanib, sorafenib, and sunitinib, can inhibit RET fusion products (32). A recent study reported that cabozantinib, an RET inhibitor, may represent a promising targeted therapy for RET fusion-positive lung ADC (33). Cabozantinib, which is FDA-approved for the treatment of metastatic thyroid cancer, may also prove to be an effective treatment for metastatic NSCLC.
FISH is generally used to detect RET and ROS1 rearrangements using RET and ROS1 Dual Color Break Apart Probes (ZytoVision, Bremerhaven, Germany) (34). IHC has also been used to detect RET and ROS1 rearrangements in NSCLC, with results that are comparable to FISH and reverse transcription polymerase chain reaction (RT-PCR) (34). Although the IHC interpretive criteria for predicting underlying rearrangements is not yet clearly defined, IHC can be used as a screening tool for further confirmatory testing.
MET
The MET gene encodes a receptor with tyrosine kinase activity (35). MET is activated by binding its ligand, hepatocyte growth factor, and regulates many physiologic processes including cellular proliferation, motility, invasion, and survival (35). The MET gene is located on the long arm of chromosome 7 at position 31, and in lung cancer, MET mutations are found in extracellular and juxtamembrane domains (36). The extracellular domain, encoded by exon 2, is required for receptor dimerization and activation (37). The presence of these mutations has been clearly defined in lung cancer; however, because of certain histologic and ethnic variations, their biologic relevance remains unclear.
In NSCLC, overexpression of MET and hepatocyte growth factor in tumor tissue is associated with higher pathologic tumor stage and worse prognosis (38), and multiple studies have reported primary MET amplification ranging from 2% to 20% in NSCLC, particularly in EGFR-TKI-naive patients (39). A recent study elucidated a mechanism of activation of MET via diverse exon 14 splicing alterations (METex14), which occurs in multiple tumor types, including NSCLC (40). The same study showed that METex14 mutations are detected more frequently in lung ADC (3%) than in SqCC (1%) (40). Importantly, in vitro studies showed sensitivity to MET inhibitors in cells harboring METex14 alterations, and patients whose tumors harbored these alterations have derived meaningful clinical benefit from MET inhibitors (40). In addition to targeting ALK and ROS1, crizotinib is also a potent MET inhibitor. Initial studies of crizotinib in patients with intermediate or high MET gene amplification demonstrated tumor responses and stable disease, which were unusually prolonged in patients with high MET amplification. Because MET amplification can also contribute to acquired resistance to EGFR-TKI therapy, combinations of MET inhibitors are being investigated in this patient population. The FDA has not yet approved any clinical tests for MET overexpression, but mutations in MET can be detected by several standard laboratory methods including FISH and PCR.
Human Epidermal Growth Factor Receptor 2
The proto-oncogene human epidermal growth factor receptor 2 (HER2), also known as ERBB2, NEU, and EGFR2, encodes for a member of the EGFR family of receptor tyrosine kinases, which plays important roles in cellular growth, differentiation, and survival. HER2 is located on chromosome 17 at position 12 (41). The HER2 protein has no ligand-binding domain and, therefore, cannot bind growth factors. However, HER2 does bind tightly to other ligand-bound EGFR family members to form a heterodimer, stabilizing ligand binding and enhancing kinase-mediated activation of downstream signaling pathways, such as those involving mitogen-activated protein kinase (MAPK) and phosphatidylinositol-3 kinase (PI3K). Activating mutations in the tyrosine kinase domain of HER2 have recently been reported in less than 5% of NSCLCs (2% of ADCs and 1% of SqCCs) (42,43). HER2 mutations in lung cancer are associated with Asian ethnicity, female sex, never-smokers, and ADC histology, particularly a lepidic histologic pattern (42,43). However, HER2 mutations can also be found in other subsets of NSCLC, including in former and current smokers (42,43). The vast majority of HER2 mutations are represented by a 12-base pair duplication/insertion of the amino acid sequence YVMA in exon 20 at codon 776 (44). The exon 20 insertion induces increased HER2 kinase activity and enhanced signaling through downstream pathways, resulting in increased tumor cell survival, invasiveness, and tumorigenicity (45).
There is no obvious association between HER2 amplification and HER2 mutation, and previous trials demonstrated no benefit of trastuzumab in HER2-amplified NSCLC. However, newer studies suggest that patients with tumors harboring HER2 insertions often respond to trastuzumab plus chemotherapy or to afatinib. A recent preclinical study demonstrated antitumor activity with the irreversible pan-HER inhibitor neratinib and the mammalian target of rapamycin (mTOR) inhibitor temsirolimus (45). Larger clinical trials are ongoing to further define efficacy of these agents in this lung cancer subtype.
Currently, HER2 testing can be carried out by several methods including IHC, FISH, chromogenic in situ hybridization (CISH), and silver-enhanced in situ hybridization (SISH). These tests are performed on tumor samples that are fixed in buffered formalin-fixed, paraffin-embedded (FFPE). A new test to measure total HER2 as well as HER2 homodimers and heterodimers is also available as a predictor of efficacy for anti-HER2 therapies (46). IHC detects HER2 protein overexpression using monoclonal or polyclonal antibodies that bind to the protein. Currently in the United States, there are two FDA-approved methods for HER2 assessment: HerceptTest™ (Dako) and HER2/neu (4B5) rabbit monoclonal primary antibody (Ventana).
BRAF
The BRAF oncogene is located on the long arm of chromosome 7 at position 34. It encodes a serine/threonine kinase that is part of the RAS/MAPK signaling pathway, a key molecular cascade that regulates important cellular functions such as proliferation, differentiation, migration, and apoptosis (47). Mutations in BRAF are more commonly seen in melanoma (50%–70%) than in lung cancer (1%–4%) (48,49). In contrast to melanoma, in which the majority of BRAF mutations occur at V600 in exon 15 of the kinase domain, BRAF mutations in lung cancer occur at other positions in exons 11 and 15 of the kinase domain, such as G469A and D594G, and they are mutually exclusive with EGFR and KRAS mutations (49). BRAF mutations in NSCLC are most frequently found in ADC and are more common in former and current smokers (49).
Clinically, BRAF inhibitors, such as vemurafenib and dabrafenib, have potent and selective activity against V600-mutant BRAF (50,51). Dabrafenib is being studied, in combination with the MAPK inhibitor trametinib, in patients with previously treated advanced NSCLC whose tumors contain the V600E mutation (50,51). Various approaches have been validated for BRAF V600 mutation detection, including Sanger sequencing, mismatch ligation assay, denaturing high-performance liquid chromatography, mutation-specific RT-PCR, IHC, next-generation sequencing (NGS), and mass spectrometry. Each method has its own sensitivity, specificity, cost, and response delay. Sanger sequencing is considered the reference test, but some data indicate that this method fails to efficiently detect BRAF mutations in many melanomas (52).
KRAS
KRAS is an oncogene located on the short arm of chromosome 12 at position 12.1. It encodes for the KRAS protein, which is involved primarily in regulating cell division as part of the RAS/MAPK pathway (53). Activating KRAS point mutations have been detected in 15% to 25% of patients with lung ADC. KRAS mutations are extremely uncommon in lung SqCC and SCLC (54). Mutations in KRAS have important cell-type-specific effects on carcinogenesis (55). The mutations found most frequently in KRAS are located at positions 12 and 13 in exon 1, and less frequently in codons 61, 63, 117, 119, and 146 (56). KRAS mutations are strongly associated with tumors in former and current smokers (57). However, they are rare in never-smokers and less common in East Asian than American and European patients (58). KRAS mutation is one of the most important predictors of resistance to EGFR-TKIs in patients with NSCLC (54). Molecular analysis has revealed that patients with activating KRAS mutations in codons 12, 13, or 61 do not derive benefit from EGFR-TKI therapy and had a 96% chance of disease progression (59,60). The current assays for KRAS genotyping include nucleic acid sequencing, allele-specific PCR methods, single-strand conformational polymorphism analysis, and probe hybridization (61).
BIOMARKERS FOR IMMUNOTHERAPY
Advances in cancer immunotherapy have generated excitement across all fields of oncology, including lung cancer. The challenges to discovering predictive biomarkers for cancer immunotherapy are significant and include the wide variety of cell types involved, multiple mechanisms of T-cell regulation, and genetic heterogeneity of tumors. Recent studies of novel agents, particularly immune checkpoint inhibitors, have shown promising results in achieving meaningful and durable treatment responses with manageable toxicity (62). New IHC markers for immune checkpoint proteins, such as programmed death-ligand 1 (PD-L1), PD-L2, OX-40, indoleamine 2,3-dioxygenase (IDO1), VISTA, and B7-H3, are being added to the pathologic analysis of NSCLC (63).
Immune checkpoints modulate the immune response to effectively balance self-tolerance and tissue destruction. Many tumors express immune checkpoints or their ligands to inhibit antitumor immune responses. One of the most important immune checkpoints is PD-L1, which is expressed in at least one-quarter of patients with NSCLC (64). Several monoclonal antibodies have been developed to block either PD-1 or PD-L1. Two PD-1 inhibitors, nivolumab and pembrolizumab, are now FDA-approved for the second-line treatment of NSCLC (65). With respect to PD-L1 inhibitors, BMS-936559, MPDL3280A, and Durvalumab are currently being evaluated in clinical trials in patients with NSCLC (63). Although several studies have demonstrated a correlation between PD-L1 expression in NSCLC cells and clinical responses to anti-PD-1 and anti-PD-L1 antibodies (65,66), some patients with tumors negative for PD-L1 expression have had a good response to immune checkpoint therapy. Recently, investigators showed that across several cancer types, including NSCLC, patients with tumors expressing PD-L1 at high levels in both malignant cells and tumor-associated immune cells (TAICs) had responses to anti-PD-L1 therapy (66). Taken together, these findings suggest that factors other than PD-L1 in the tumor microenvironment, including tumor-infiltrating lymphocytes (TILs) and tumor-associated macrophages (TAMs) (67,68), may drive responses to anti-PD-1 and anti-PD-L1 therapies.
The presence or absence of TILs and PD-L1 has promoted a new tumor stratification system based on the tumor microenvironment (68). This stratification classifies tumors into four categories: type I or adaptive immune resistance (TIL+/PD-L1+), type II or immunologic ignorance (TIL–/PD-L1–), type III or immunologic tolerance (TIL+/PD-L1–), and type IV or intrinsic induction (TIL–/PD-L1+) (68). The clinical validation of such a tumor stratification scheme for immunotherapy will encourage pathologists to incorporate new tools, such as multispectral immunofluorescence analysis of immunologic markers, into clinical practice.
MOLECULAR DIAGNOSTIC ASSAYS
The earliest approaches to molecular diagnostics in lung cancer were characterized by the use of a combination of assays, such as IHC, Sanger sequencing, RT-PCR, and FISH, that each interrogated genomic changes in a specific gene. These assays have led to a better understanding of disease pathogenesis and the development of personalized medicine in lung cancer. Newer methods, including multiplex hot spot mutational testing and NGS, have the capability of providing broader molecular profiling to define the full genomic context of an individual tumor.
Immunohistochemistry
Numerous studies have examined the use of specific antibodies for IHC detection of known driver oncogenes. IHC provides a rapid assessment of ALK or ROS1 protein expression as an initial screening method before FISH testing (Figure 3.5) (31). These antibodies are designed to detect wild-type ALK or ROS1 proteins based on the concept that the majority of tumors harboring recurrent rearrangements involving ALK or ROS1 will have elevated levels of expression of the corresponding protein. Several ALK and ROS1 IHC antibodies have demonstrated good sensitivity and specificity when compared to FISH (69,70). In contrast, screening for RET fusions with IHC has not been successful.
Sanger Sequencing
Direct DNA sequencing after PCR-based amplification was one of the first methods used to detect mutations in lung cancer, such as those involving KRAS and EGFR. The procedure involves a single-stranded DNA template and DNA extension, via DNA polymerase, from a bound primer using standard deoxynucleotides. DNA fragments are then exposed to capillary electrophoresis and fluorochromes are used for automated sequence analysis (71). This method was previously used extensively in clinical pathology, but has now been replaced by multiplex or high-throughput assays such as reverse transcription polymerase change reaction, fluorescence in situ hybridization, multiplex hot spot mutational testing, and next-generation sequencing.
Reverse Transcription Polymerase Chain Reaction
The RT-PCR method is used for the detection of gene fusions in RNA extracted from a patient’s tumor (31). Gene expression is detected via the creation of complementary DNA (cDNA) transcripts from mRNA and the amplification of cDNA is measured quantitatively with the use of fluorescent probes (72). Quantitative RT-PCR is considered the gold standard for measuring the number of copies of specific cDNA targets. The extensive use of this technique has resulted in the development of various protocols that enable the generation of quantitative data using fresh, frozen, or archived paraffin samples from whole-tissue biopsies, microdissected samples, single cells, or tissue-cultured cells (73). The advantages of this method include a rapid processing time and the requirement for a small number of cells. However, RT-PCR is specific for particular fusion gene pairings and will not detect alternate partners. Other limitations include the high level of technical skill required to perform the assay and the need for high-quality RNA (74).
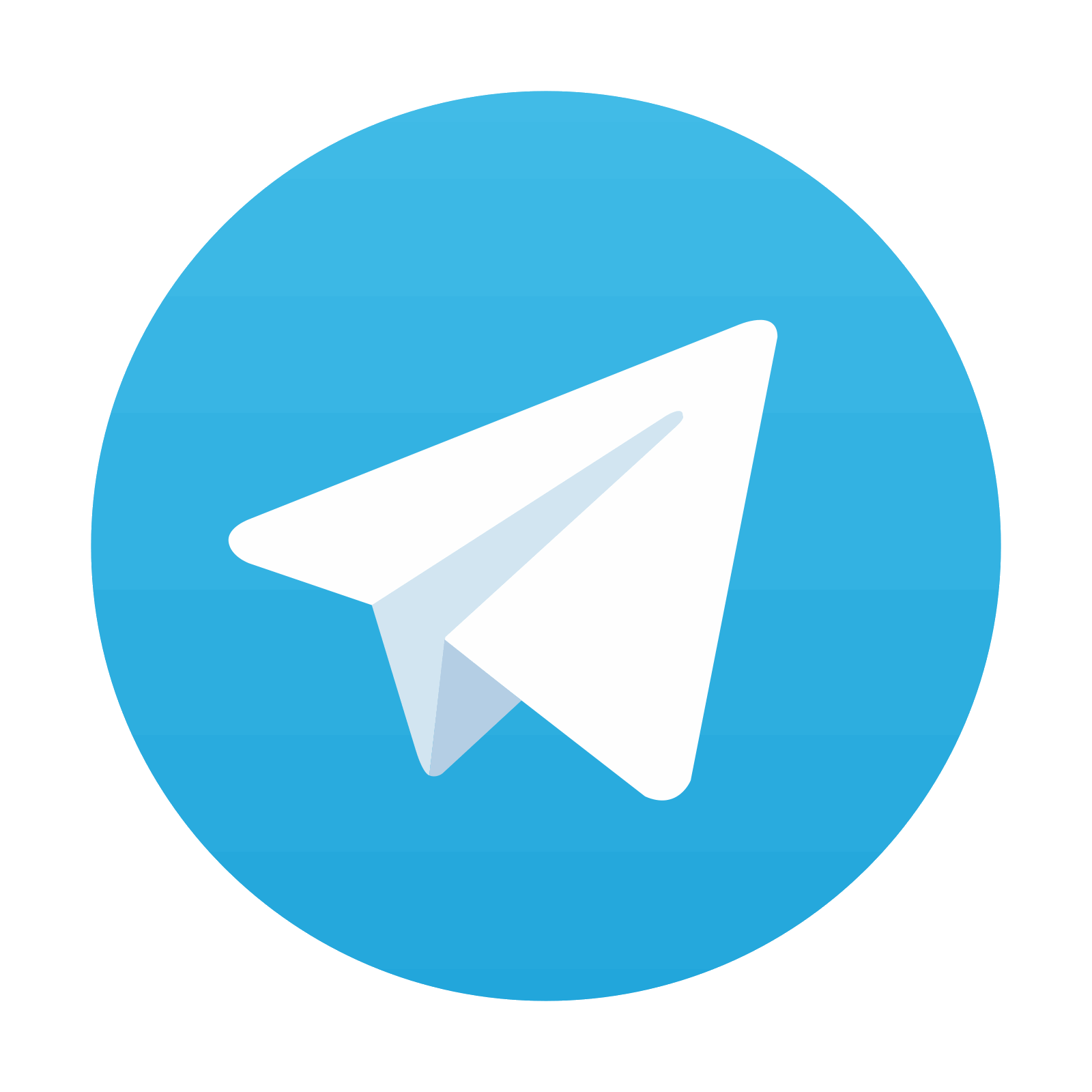
Stay updated, free articles. Join our Telegram channel
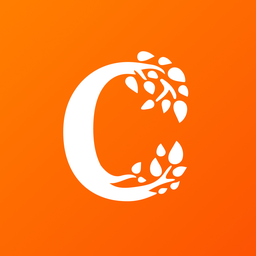
Full access? Get Clinical Tree
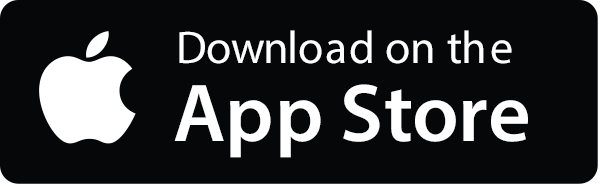
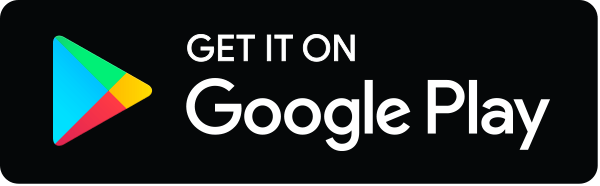