Lipids
Cholesterol
Ceramide
Sphingomyelin
Diacylglycerol (DAG)
Cis-unsaturated phospholipids
Phosphatidylserine
Phosphatidylethanolamine
Phosphatidylinositol
Phosphatidylinositol bi-phosphate (PIP2)
Protein
Caveolins (-1, -2, and -3)
G-proteins
G-alpha, -G-beta
Adrenergic receptor (AR)
Beta-1, beta-2, beta-3?
Cytokine receptors
Epidermal growth factor receptor (EGFR)
Platelet-derived growth factor receptor (PDGFR)
Insulin receptor
Bradykinin receptor
Endothelin receptor
GRB-2–growth factor receptor bound protein 2
SOS–son of sevenless
Signal tranduction
Protein kinase C (PKC) alpha subunit
Phosphatidylinositol 3-kinase (PI3K)
Mitogen-activated protein kinase (MAPK)
eNOS, nNOS
Calmodulin
Cytoskeletal
Actin
Myosin
Ezrin
Caveolins: The Markers for Caveolae
Caveolins not only are the main elements of caveolae, but they are the “corner-stone” of these lipid rafts.
Caveolins were identified quite fortuitously at the end of 1980s, when investigators studying chicken embryonic fibroblast transformed with Rous Sarcoma Virus (RSV) purified several phospho-tyrosine containing proteins, one of those being resistant to nonionic detergents extraction [27]. This 22-kDa protein, later identified in caveolae by EM analysis and thus termed caveolin, showed an immunohistochemical pattern consistent with parallel arrays of individual vesicles along the actin stress fibers, and with altered localization after cellular transformation [28]. The latter observation supported the hypothesis that the phosphorylation of caveolin occurred upon transformation with v-Src, suggesting the hypothesis of a possible role of caveolin and caveolae in oncogenesis [28].
In 1992, studies on cellular trafficking identified caveolin (also called VIP21) to localize to the Golgi apparatus, the plasma membrane, and membrane-bound vesicles [29].
Caveolin Genes and Their Products
Caveolin-1 and -2
Caveolins is the general term to define the three members of the three caveolin (CAV1-3) gene family identified so far, each encoded by a separate gene (Fig. 18.1). The gene coding for caveolin-1 (CAV1), is the first gene to be identified, and is composed of three exons that are highly conserved in sequence and structure across species, while the gene coding for caveolin-2 (CAV2) was discovered by protein micro-sequencing of purified adipocyte caveolae membrane domains in which the polypeptide revealed a remarkable similarity to caveolin-1, but differing in some key conserved caveolin-1 residues [30].
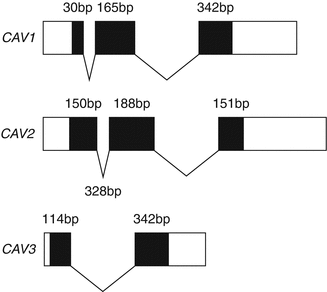
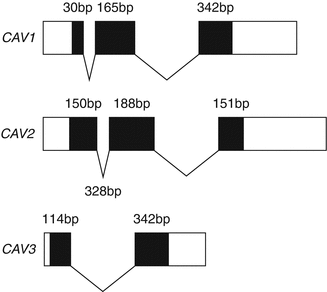
Figure 18–1.
Schematic depiction of the caveolin gene family. Black boxes indicate the exon coding sequence for each caveolin family member. The numbers indicate the number of coding sequence nucleotides in each exon
Finally, using the sequence of CAV1 as probe, the group of Michael P Lisanti cloned the gene coding for caveolin-3 (CAV3), and characterized its expression to be mainly, if not exclusively muscle-specific [31].
The CAV1 and CAV2 genes are expressed almost ubiquitously, and in particular, they are co-expressed in most differentiated cells types, especially in adipocytes, endothelial cells, fibroblasts, smooth muscle and type I pneumocytes, but they were initially believed to be absent in mature striated muscle except skeletal muscle satellite cells [32, 33].
However, in the last several years, increasing literature brought forth strong evidence of caveolin-1 expression in both atrial and ventricular cardiomyocytes, although at variable levels and localization [34]. In addition, it appears that caveolin-1 and caveolin-3 interact and form heterooligomeric complexes in atrial cardiomyocytes [35]. Unfortunately, the complexity of the caveolin family took a further step with the discovery of multiple isoforms for both caveolin-1 and -2. Caveolin-1 presents with two isoforms, termed α and β; caveolin-1α consists of residues 1–178, while caveolin-1β, originating from an alternate translation initiation site occurring at a methionine in position 32, contains residues 32–178, resulting in a protein ∼3 kDa smaller in size [32]. Although shorter than caveolin-1α, caveolin-1β represents a functional isoform capable to drive caveolae formation similarly to caveolin-1α in Drosophila melanogaster Sf21 cells, which lack endogenous caveolae [36].
The functional significance of these distinct caveolin-1 isoforms remains uncertain, although caveolin-1α appears to be localized primarily to deeply invaginated caveolae and more efficiently drive the formation of caveolae than caveolin-1β [20, 37].
Three isoforms have been identified for caveolin-2, the full-length caveolin-2α and two alternate splice variants, called caveolin-2β and caveolin-2γ, which show distinct subcellular distribution from caveolin-2α, although their functional significance is largely unknown [6].
Caveolin-3
The tissue distribution of CAV3 has been intensely studied in mouse, in which CAV3 expression appears to be restricted to differentiated skeletal and cardiomyocytes, while it is absent in non-proliferating C2C12 skeletal murine myoblasts compared to the proliferating precursor myoblasts, which express both caveolin-1 and -2 [38]. In addition, caveolin-3 levels are intimately associated with muscle development, and experiments performing the differentiation of C2C12 skeletal myoblasts in culture showed CAV3 up-regulation [39], while treatment with a CAV3-antisense prevented myotube fusion in vitro [40].
Caveolin-3 has been shown to function similarly to caveolin-1, with which it shares high protein homology. In fact, caveolin-3 is connected to the sarcolemma and modulates the function of the dystrophin glycoprotein complex (DGC), the major protein ensemble linking the contractile apparatus to the plasma membrane in striated muscle and associated with a variety of muscular dystrophies with cardiac involvement and primary heart diseases all presenting with frequent arrhythmias [41–43]. Dystrophin, as well as several members of the DGC including α-sarcoglycan and β-dystroglycan, cofractionate with caveolin-3 in cultured mouse C2C12 myocytes [39]. In addition, Co-IP experiments demonstrated that dystrophin forms a stable complex with caveolin-3 and that dystrophin and caveolin-3 co-localize [44]. Furthermore, since the WW-like domain of caveolin-3 binds the C-terminus of β-dystroglycan, a region containing a PPXY motif it is possible that caveolin-3 competes with dystrophin for the binding to β-dystroglycan; this will inhibit dystrophin binding with subsequent reduced dystrophin presentation to the sarcolemma [44]. In fact, in muscle biopsies from patients suffering from Duchenne Muscular Dystrophy (DMD) with progressive muscle weakness, respiratory failure and the development of DCM associated with arrhythmias, caveolin-3 is shown to be up-regulated along with an increased number and size of caveolae on the plasma membrane [45, 46]. Similarly, caveolin-3 overexpression and increased caveolae number and size occur also in dystrophin-deficient mdx mouse [46, 47].
Caveolins and Animal Models
One of the first experimental approaches to study the unknown role of a known protein is to generate an animal model in which the target gene has been ablated. Knockout mice have been generated for all known caveolins, but only the CAV1 -/- and CAV3 -/- mice have been particularly interesting in studying caveolin function in the biology of the myocardium.
It is interesting to note that all the caveolin deficient mouse models generated (CAV1 -/-, CAV2 -/-, CAV3 -/-, CAV1/3 -/- double knockout mice) are viable and fertile despite the tissue distribution and the involvement in such a variety of biological processes observed for caveolins.
The CAV1 -/- Mice
Two independent groups generated CAV1 null mice. Drab et al., in 2001 showed that upon targeted disruption of CAV1, the animals showed absence of caveolae in all tissues usually expressing caveolin-1 and leading to impaired nitric oxide and calcium signaling in the cardiovascular system, causing aberrations in endothelium-dependent relaxation, contractility, and maintenance of muscular tone [48]. In addition, the lungs of CAV1 -/- mice exhibited alveolar thickening due to unrestrained endothelial cell proliferation and fibrosis [48].
In the same year, Razani and colleagues generated viable and fertile caveolin-1 null mice demonstrating the same caveolar aberration previously affirmed and showing that absence of caveolin-1 could not prevent normal caveolae to be formed in tissues expressing caveolin-3 such as skeletal and cardiac muscles [49]. In addition, the CAV1 -/- mouse demonstrated an almost complete loss of caveolin-2 confirming the role of caveolin-1 in hetero-oligomerization with caveolin-2, and the ability of caveolin-1 to recruit caveolin-2 from the Golgi to the plasma membrane [48, 49].
The recent identification of CAV1 expression in cardiomyocytes supports the observed development of cardiac hypertrophy and cardiomyopathy in CAV1 -/- animals with reduced lifespan due to sudden cardiac death (SCD) [50–52]. In addition, the cardiac hypertrophy and collagen deposition in caveolin-1-deficient mice are due to the deficient inhibitory effect of cavoelin-1 on the transforming growth factor-β1 (TGFβ1) signaling, a pivotal mediator of cardiac hypertrophy [53].
The CAV3 -/- Mice
The caveolin-3 null mouse demonstrates the absence of muscular caveolae, while expression of caveolin-1 and -2 was normal in non-muscle cells [54, 55]. The animals developed muscular dystrophy phenotype similar to limb girdle muscular dystrophy (LGMD) observed in patients with LGMD-1C. In addition, CAV3 -/- demonstrates a cardiomyopathy phenotype similar to that described above in CAV1 -/- animals.
Using cardiac gated MRI and TEE Woodman et al., found that, at 4 mo of age, CAV3 -/- showed significant cardiac hypertrophy and reduced fractional shortening [56].
Cardiac histological analysis revealed perivascular fibrosis, myocyte hypertrophy, and cellular infiltration, derived from the exclusion of the DGC from lipid rafts and hyper activation of Ras-p42/44 MAP kinase cascade in CAV3 null cardiac tissue [56]. These changes are consistent with the known role of p42/44 MAP kinase activation in cardiac hypertrophy and the role of caveolin-3 as a negative regulator of the Ras-p42/44 MAP kinase cascade, similar to that of caveolin-1 [56].
Interestingly, the caveolin-3 T63S mutation has been recently identified in patients with HCM and DCM, although the exact pathological mechanism is unknown [57].
The CAV1/3 -/- Mice
The generation of truly caveolae-deficient animals was accomplished by interbreeding Cav-1 and Cav-3 null mice, to produce caveolin-1/3 double knockout mice (Cav-1/3 dKO). Surprisingly, Cav-1/3 dKO mice are viable and fertile, despite a complete absence of morphologically identifiable caveolae in muscle and nonmuscle cells [58]. Additionally, these mice are deficient in the expression of all three caveolin family members, as caveolin-2 is unstable and degraded in the absence of caveolin-1.
The double KO present with a severe cardiomyopathy phenotype, already evident at 2 months of age with more pronounced left ventricular wall thickness and ventricular septum thickness accompanied by dramatic cardiac hypertrophic, interstitial inflammation, perivascular fibrosis, and myocyte necrosis [58]. Thus the combined ablation of both caveolin-1 and -3 profoundly alter cardiac structure and function leading to a remarkable risk of SCD.
Caveolins: The Biochemistry
Caveolins Protein Domains
Regardless of the isoform variability, all three caveolin proteins contain some protein motifs highly conserved throughout evolution such as the eight amino acids FEDVIAEP, which constitutes the “caveolin signature sequence” and is localized proximal to the N-terminus of the protein [6].
Another characteristic protein domain present in caveolins is the “scaffolding domain”, a motif proximal to the hydrophobic transmembrane domain, which interacts with different signal transduction molecules (Fig. 18.2) [59, 60]. Although researchers recognize the importance of each protein domain present in caveolins, it appears that proper caveolin function such as lipid raft association, extrusion from the Golgi apparatus and caveolae targeting, greatly if not solely depends on the overall protein folding and tertiary structure [61].


Figure 18–2.
Prototypic caveolin structure and critical protein motifs
Despite the high amino acids sequence similarity among the different caveolins, human caveolin-1 and-3 share the highest protein homology with ∼65 % identity and ∼85 % similarity, while caveolin-2 shares only ∼38 % identity and ∼58 % similarity to human caveolin-1. This remarkable resemblance between caveolin-1 and -3 and their divergence from caveolin-2 may explain why both caveolin-1 and -3, but not caveolin-2 can form caveolae alone or in association with other caveolins in cells lacking caveolae structures such as MDCK and epithelial CaCo-2 cells [62]. In addition, caveolin-1 and -3, but not caveolin-2 undergoes an irreversible post-translational modification inserting a palmitoyl acid molecule on a cysteine residue at their C-terminus [63]. It is believed that palmitoylation of caveolin-1 and -3 stabilizes the caveolin oligomers and increases the association with the membrane through hydrophobic domains [64, 65].
The expression of both caveolin-1 and -3 is also necessary to stabilize caveolin-1/-2 and caveolin-3/-2 heterodimers and to ensure the correct membrane localization of caveolin-2 [66].
Caveolins Protein Topology
Both the N- and the C-termini of the prototypical caveolin are localized to the cytoplasm, while the central hydrophobic domains are “embedded” in the plasma membrane, and no extracellular segments were detected (Fig. 18.3) [67]. This spatial conformation allows the N-terminal phosphorylation of caveolin-1 by the α subunit of Protein Kinase C (PKCα) and, as previously mentioned, the palmitoylation at the C-terminus of both caveolin-1 and -3 [36, 63]. The ultimate protein structure and topology of caveolins originates in the endoplasmic reticulum (ER), where caveolins are believed to be introduced in the plasma membrane and form a hairpin loop configuration through a transmembrane domain containing 32 hydrophobic amino acids (residues 102–134), which precludes caveolins to completely extend across the plasma membrane [68].
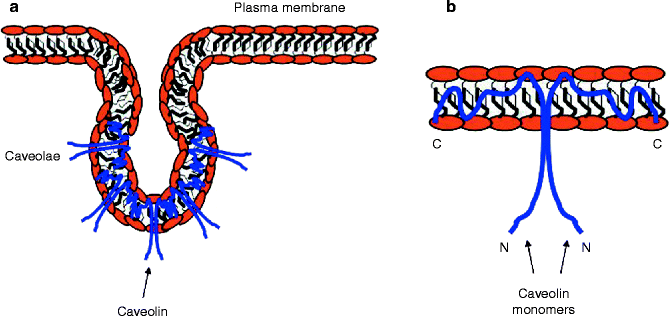
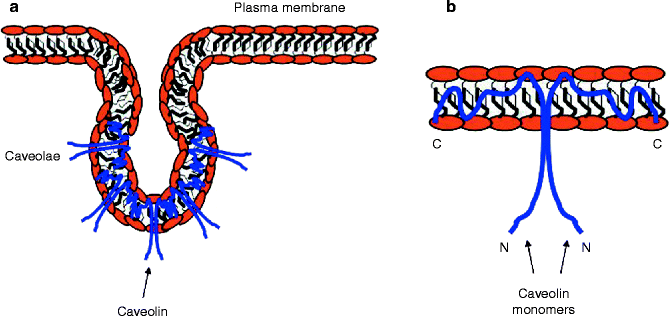
Figure 18–3.
Caveolae and caveolin topology. (a) Caveolae form plasma membrane invaginations that differ from the clathrincoated endocytic vesicles. (b) Caveolins exhibit both N- and C-terminus ends into the cytoplasm, although their central portion is tightly embedded into the plasma membrane, where most of the interactions with ion channels are hypothesized to occur. Caveolin topology dramatically determines the role of these proteins in lipid raft internalization and functional modulation of its binding partners
In addition, the scaffolding domain (residues 82–101) proximal to the N-terminus and the segment 135–150, proximal to the C-terminus are tightly connected to the membranes [6]. Caveolin-1 constructs containing residues 1-101 are sufficient for membrane localization, while the segment 1-82 remained in the cytoplasm [69]. It has been established that the (KYWFYR) motif was sufficient to confer membrane localization to a GFP fusion protein [70]. In addition, residues 135–150 include a unique Golgi-targeting sequence, and a construct including only this segment of caveolin shows a prevalent Golgi localization [71, 72].
Caveolin-1 contains an oligomerization domain (residues 61–101), which promotes the homo-oligomerization of up to 16 individual caveolin-1 molecules [59], while a second stage of oligomerization occurs during transport from the trans-Golgi to the plasma membrane thus forming a large network of caveolin [73]. In contrast, caveolin-2 is unable to generate large homo-oligomeric complexes and so to develop caveolae by itself [30], while it can form hetero-oligomers with caveolin-1 in the ER to stabilize caveolin-2 against proteasomal degradation and consent to its transport from the Golgi apparatus to the plasma membrane [49, 74–76].
Similarly to caveolin-1, caveolin-3 forms large homo-oligomeric complexes in striated muscle, and co-immunoprecipitation (Co-IP) and membrane co-fractionation studies in rat neonatal cardiomyocytes demonstrated that caveolin-3 associates with caveolin-2 in hetero-oligomers as it occurs for caveolin-1/-2 [77, 78].
Caveolae: The Function
Caveolae and Lipids Regulation
Due to their high lipid content, it is not surprising that caveolae are involved in lipid metabolism, in particular in the regulation of cholesterol.
Studies employing cholesterol-binding agents such as filipin and nystatin resulted in the disruption of caveolae, suggesting the importance of cellular cholesterol balance on caveolar structure [26]. In addition, increase in cholesterol levels up-regulates caveolin-1 expression through specific binding to two steroid regulatory elements (SRE) on the caveolin-1 promoter, while decreased cholesterol levels results in CAV1 down-regulation [79, 80]. Moreover, oxidation of cholesterol into cholesterone by cholesterol oxidase alters the cellular cholesterol balance and results in caveolin-1 internalization from the plasma membrane to the ER and the Golgi apparatus [81].
Caveolin-1 transports newly synthesized cholesterol from the ER to membrane caveolae, and than to plasma high-density lipoproteins (HDL), while extracellular cholesterol primarily enters cells via clathrin-mediated endocytosis of low density lipoproteins (LDL) [82]. Therefore, caveolae may represent the principal location for cholesterol exchanged between HDL and the cell membrane.
Caveolae and Endocytosis
Caveolae are believed to play a role in endocytosis, oncogenesis and internalization of pathogenic bacteria [83].
Caveolae represent a clathrin-independent mechanism of endocytosis for the turnover of adhesive complexes, and since their discovery, caveolae were believed to play a role in endocytosis owing that they protrude from the plasma-membrane into the cytoplasm. However, there are conflicting evidences involving caveolae in constitutive endocytic trafficking. Caveolin-1 cloned as N- and C-terminus GFP fusion protein and expressed in HeLa, A431, and Madin-Darby canine kidney (MDCK) cells demonstrated that caveolae require both cholesterol and an intact actin cytoskeleton to maintain their integrity [84]. This may support the hypothesis that caveolae are intimately connected to the actin network. Conversely, Pelkmans and colleagues demonstrated that caveolae play an instrumental role in the internalization of SV40 viral particles, and that this internalization was triggered by molecular signals from the virus, able to activate the caveolae otherwise “dormant” in the stable state previously described [85].
Caveolae and Signal Transduction
Caveolae have been recognized to play an essential role in signal transduction since biochemically purified caveolae contained multiple signaling molecules, including heterotrimeric G proteins, which in the myocardium are involved in sympathetic tone response though β-adrenergic receptors (β-AR) [86, 87]. In addition, caveolae mediate other signaling factors such as H-Ras, Src-family kinases, and endothelial nitric oxide synthase (eNOS) [6].
Recently, it was shown that caveolae integrity and both caveolin-1 and caveolin-3 can modulate atrial natriuretic peptide (ANP) and brain natriuretic peptide (BNP) production, along with Ras-p42/44 MAP kinase cascade (MEK and ERK) and Akt phosphorylation activity [52, 87]. In addition, caveolae have also been implicated in the modulation of cardiac hypertrophy [88].
The finding of such concentration of signaling molecules in caveolae suggests that caveolins act as scaffolding proteins, through the amino acids 82-101 motif, to concentrate and localize these elements for a rapid and specific cell response.
Caveolae and Ion Channels
Being highly expressed in excitable cells of the nervous system, skeletal muscle and myocardium, there is no surprise to find that a variety of ion channels are localizing to caveolae (Table 18.2). However, it took more than a decade from the first report identifying caveolins in caveolae to obtain evidence that these plasmalemmal vesicles not only cluster and scaffold a plethora of cell membrane proteins including signaling molecules, but also anchor, distribute, transport and possibly modify ion channels on the cell surface.
Table 18–2
Cardiac ion channels localizing to caveolae
Cardiac ion channels |
---|
Cardiac sodium channel (Nav1.5) [89] |
Voltage-gated Kv1.5 [92] |
Pacemaker channel HCN4 [93] |
Na/Ca2+ exchanger (NCX) [94] |
ATP-sensitive K+ [KATP] (Kir6.1) [95] |
Volume-regulated anion channel (VRAC) [96] |
Transient receptor potential canonical 1 (TRPC1) [97] |
In 2000, Martens and colleagues identified Shaker-like K+ channels (Kv2.1) to non-caveolar lipid rafts [98], although the role of these vesicles in Kv2.1 function regulation was elucidated a year later by the same laboratory, showing that cyclodextrin treatment not only led to cholesterol depletion, but also caused a significant shift in steady-state inactivation of the KV2.1 channel [99]. This first evidence that ion channel localize to lipid rafts suggested that other ion channels may be present in caveolae and that caveolins may regulate ion channels function.
In fact, in 2001 Martens and colleagues localized the voltage-gated potassium channel Kv1.5 to caveolae in transfected fibroblasts [92], and later they showed that caveolins are involved in regulating Kv1.5 trafficking to cholesterol-rich membrane microdomains [99]. It is interesting to note that Kv1.5 binds the major protein of the Z-line, α-actinin-2 [100], which is connected to the actin network supporting the concept that caveolae interact with the cytoskeleton (Fig. 18.4).
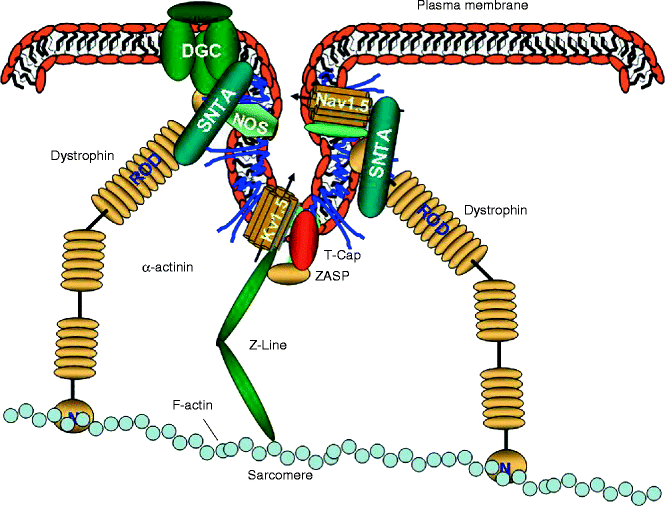
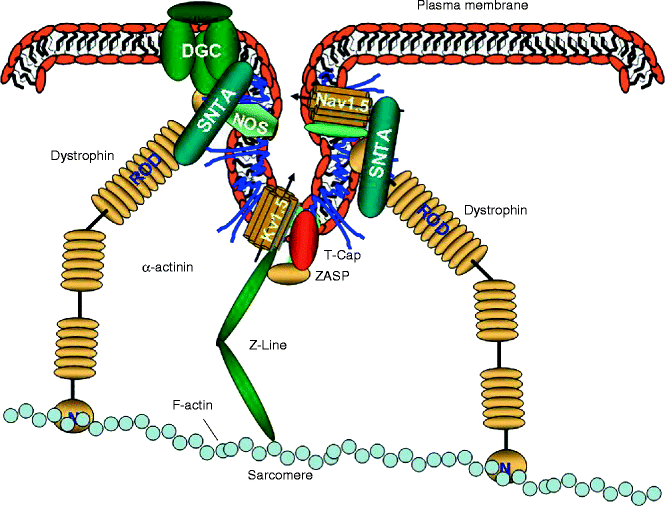
Figure 18–4.
Cytoskeleton, caveolae, and caveolins. Caveolae are intimately associated with the cytoskeleton of the cardiomyocytes, and are ultimately linked to the contractile apparatus of the myocardial cells. DGC dystrophin glycoprotein complex, SNTA α1-syntrophin
The Kv1.5 channels are expressed in the intercalated disk of human cardiomyocytes and are associated with connexin 43 and N-cadherin [101]. Cytoskeletal alteration using cytochalasin D, which disrupts the filament actin (F-actin), leads to a massive increase in I K+, while the phenomenon was totally abolished when the cells were by pre-incubated with phalloidin, an F-actin stabilizing agent [100].
Probably the most intriguing discovery concerning the relationship between cardiac ion channels and caveolae was that the SCN5A-coded voltage-gated Na+ channels (Nav1.5) have been reported to localize to ‘caveolin-rich membranes’ in cardiac myocytes [89]. In addition, the α-subunit of the L-type Ca2+ channel (Cav1.2) was originally found in caveolin-enriched membranes in smooth muscle [102].
It is interesting to note that mutations in both Nav1.5 and Cav1.2 have been associated with a primary arrhythmogenic disorder such as the long QT syndrome (LQTS) in human subjects [103–105].
Similarly, skeletal muscle caveolae have been found to contain also the 1,4-dihydropyridine receptor (DHPR or RYR) in the sub-sarcolemmal region of the myofibers [106]. In addition, caveolin expression induces the Cl2 channel function [97].
It is hypothesized that ion channels, which localize to caveolae probably through the scaffolding domain of caveolin-3, reach these lipid rafts after post-translational modifications such as palmitoylation and myristoylation, or by glycophosphatidylinositol (GPI) membrane anchors, mostly occurring in the Golgi apparatus [107].
It is still unclear whether caveolin, which can independently drive caveolae formation, can organize membrane lipids and stabilize transient membrane rafts, so that channel proteins might perform as a focal point in raft development.
Alternatively, the association between ion channels and caveolae, may not occur through protein/lipid interactions but rather through protein/protein interactions with the direct involvement of caveolins such as caveolin-3, or the direct binding of other caveolin-associated proteins such as the PDZ motif containing protein PSD95 (postsynaptic density protein 95), which has been reported to associate with low-density lipid rafts in mammalian cells [108]. However, the PDZ protein domain may be only one player in the localization of ion channels to raft domains. In fact, Kv2.1 channels do not contain standard PDZ binding sequences [109], and removal of the PDZ binding motif from the Kv1.5 channel does not prevent its lipid raft association [92].
Caveolae, the Cytoskeleton and Ion Channels
It is interesting to note that many key structural elements, such as the Z-band alternatively spliced PDZ motif protein (ZASP), signaling molecules such as eNOS, and Nav1.5 modulator, such as α1-syntrophin (SNTA) contain a PDZ domain, and both eNOS and SNTA also localize to caveolae and directly bind caveolin-3 [110–115]. In addition, all the aforementioned proteins localize or are associated with the sarcolemma via direct or indirect interaction with the large protein dystrophin and the dystrophin-associated glycoprotein complex (DGC), thus modulating ion channel regulation [41–43].
Caveolin-3, the Cytoskeleton and Ion Channels
Syntrophins are known to contain two pleckstrin homology (PH) domains, a PDZ domain, and a syntrophin-unique (SU) at its C-terminus. Syntrophins directly binds dystrophin through its PH domain distal to the N-terminus and the highly conserved SU domain [114, 116]. The PH domain proximal to the N-terminus and the PDZ domain interact with other membrane components such as phosphatidyl inositol-4, 5-bisphosphate (PIP2) [117], neuronal NOS (nNOS) [118], aquaporin-4 [119], stress-activated protein kinase-3 [120], and Nav1.5 [121], thereby linking all these molecules to the dystrophin complex (Fig. 18.4) [122].
In addition, syntrophins bind the C-terminus of Nav1.5 through its PDZ domain, regulating the gating properties of the sodium channel [123].
Remarkably, caveolae not only cluster syntrophins, but caveolin-3 directly binds syntrophin [113] as well as other important focators such as the Na+/Ca2+ exchanger [124], the L-type Ca2+ channel [113], eNOS and nNOS, and the DGC (Fig. 18.4) [110–112]. Therefore, the association of caveolin-3 with α1-syntrophin, through its binding to F-actin, nNOS, and Nav1.5, appears to be involved in regulating structural and electrical functions as well as signal transduction in heart failure [113]. Recently, the p. A257G mutation in the α1-syntrophin-encoding gene SNTA1 was identified in LQTS [125]. Electrophysiological analysis in murine cardiomyocytes when Nav1.5 was co-expressed with mutant SNTA1 identified that the mutant SNTA1 had a profound effect on the sodium current causing an increased peak current densities and a shifted onset and peak of the currents toward more negative potentials, as well as a delay of sodium current decay [125]. In addition, coimmunoprecipitation assay demonstrated that human SNTA1 can physically interact with Nav1.5 and that perturbations in SNTA1 might alter Nav1.5 function [125]. Interestingly, Nav1.5 function can also be affected through post-translational modification. In fact, SNTA1 binds nNOS to the nNOS inhibitor plasma membrane Ca-ATPase subtype 4b (PMCA4b), and the p.A390V mutation in SNTA1 identified in LQTS subjects selectively disrupts the association of PMCA4b with the nNOS/SNTA1/Nav1.5 complex leading to the released of nNOS inhibition by PMCA4b and causing increased direct nitrosylation of the sodium channel [126]. The increased nitrosylation of Nav1.5 by p.A390V-SNTA1 causes an increased peak and late sodium current compared with the wild-type SNTA1, while this increase was partially inhibited by NOS blockers. This effect was consistent with the characteristic biophysical dysfunction for sodium-channel-mediated LQTS (LQT3) [126].
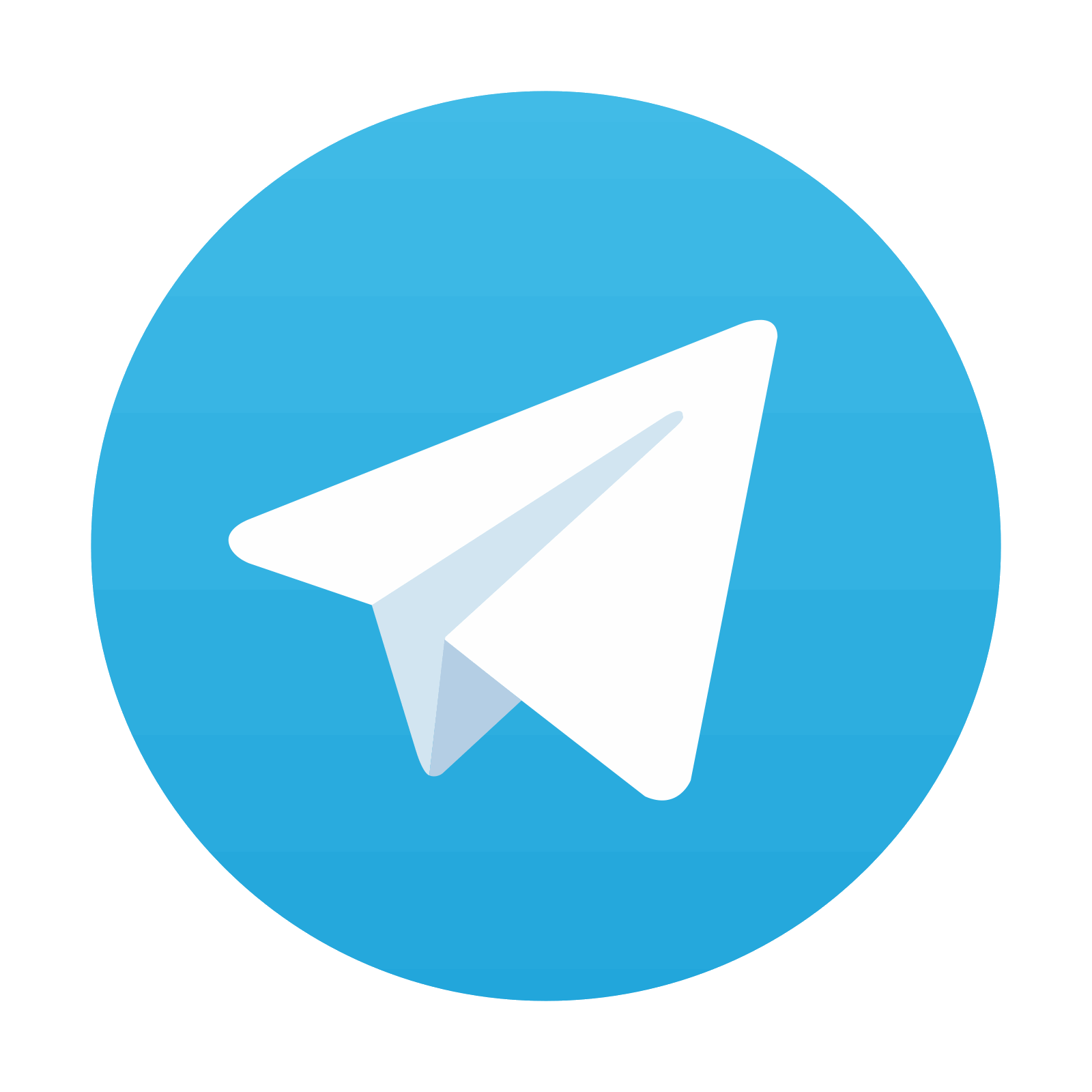
Stay updated, free articles. Join our Telegram channel
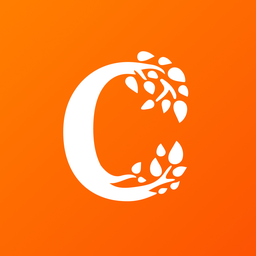
Full access? Get Clinical Tree
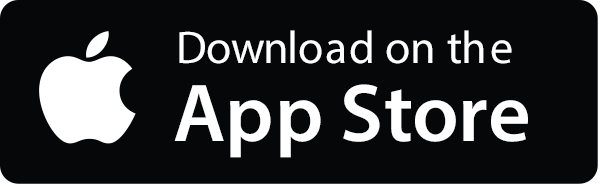
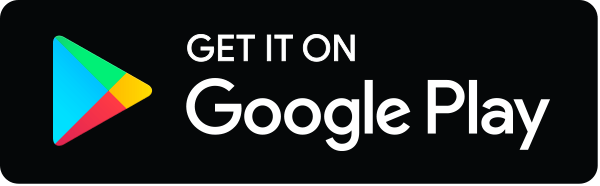