, Dilip R. Karnad2 and Snehal Kothari3
(1)
Cardiac Safety Services Quintiles, Durham, North Carolina, USA
(2)
Research Team, Cardiac Safety Services Quintiles, Mumbai, India
(3)
Cardiac Safety Services Global Head, Cardiac Safety Center of Excellence Quintiles, Mumbai, India
The heart is a supreme example of biomechanical engineering that, under normal circumstances, operates with elegant economy (Turner 1994).
3.1 Introduction
This chapter provides an overview of healthy cardiovascular structure and function, followed by an overview of cardiac pathophysiology and disease. In each case, attention falls first on the heart, since multiple subsequent chapters focus on proarrhythmia. Nonetheless, discussions of the cardiovascular system are also pertinent to topics addressed later in the book.
The heart is a supreme example of biomechanical engineering, and, under normal circumstances, it operates with elegant economy (Turner 1994). As acute metabolic demand varies with increases and decreases in skeletal muscular activity, cardiac activity adapts commensurately, enabling skeletal musculature to receive appropriate supplies of blood, and hence oxygen, to facilitate its physical activity. This intimate and biologically sensible arrangement is complemented by blood flow within the systemic vasculature, which (under normal circumstances) ensures adequate oxygen supply to all bodily tissues.
3.2 The Heart
The human heart is a dual muscular pump: both the left side and the right side have an upper chamber, or atrium, and a lower chamber, or ventricle. Blood flowing through the cardiovascular system distributes oxygen (and other nutrients) to cells and also collects carbon dioxide. Deoxygenated blood containing carbon dioxide returns to the right atrium and is sent via the right ventricle to the lungs. Carbon dioxide is removed from the blood, which is also reoxygenated. The oxygen-rich blood flows to the left atrium, from which it passes into the left ventricle, which ejects it into the aorta. Blood is then distributed through the body via arteries, arterioles, and body tissue capillaries, where exchange of oxygen and carbon dioxide occurs. Blood then flows back to the heart via capillaries, venules, and veins.
The heart muscle, or myocardium, surrounds both sides of the heart and influences the flow of blood through all four heart chambers by its regular pattern of squeezing and then relaxing. Valves within the heart control the flow of blood in the desired directions (each atrium to the respective ventricle and from each ventricular into the respective artery). The locations of heart valves are as follows:
The tricuspid valve is between the right atrium and right ventricle.
The pulmonary valve is between the right ventricle and the pulmonary artery.
The mitral valve is between the left atrium and left ventricle.
The aortic valve is between the left ventricle and the aorta.
A specialized network of blood vessels called the coronary circulation supplies the heart. Coronary arteries branch off the aortic root just distal to the attachments of the aortic valve leaflets and are therefore the first arteries to branch off the aorta. The right coronary artery supplies oxygen to the right side and usually the bottom of the heart. The left coronary artery divides into two branches, the anterior descending branch and the circumflex branch. The anterior descending branch supplies blood to the left side of the heart and the interventricular septum, and both the anterior descending branch and the circumflex branch supply blood to the lateral aspect and rear of the heart. By curling around the heart and dividing into smaller and smaller branches, the coronary arteries are able to supply every muscle fiber in the myocardium with blood and, hence, oxygen (Turner and Durham 2009).
3.2.1 The Cardiac Cycle and the Action Potential
The discipline of cardiac electrophysiology studies how the cardiac conduction system facilitates the flow of electrical stimuli across cardiac tissues. The discipline is employed in clinical practice for the diagnosis of cardiac rhythm abnormalities and pathophysiology and can also be used in proarrhythmic cardiac safety evaluations: our attention will focus on the latter.
The electrocardiogram (ECG) is a well-known pattern of electrical activity. For present purposes, a simplified approach can be adopted, with focus falling on just one of the multiple intervals that can be identified on an ECG. Figure 3.1 is a stylistic representation of an ECG waveform, with the landmarks and interval of interest identified. These are the onset of the QRS complex, the offset of the T-wave, and the distance between these two points, known as the QT interval. Given that drug-induced prolongation of the QT interval is of considerable importance in the book’s discussions, a stylistic representation of QT interval prolongation is shown in Fig. 3.2.



Fig. 3.1
Stylistic representation of the ECG waveform

Fig. 3.2
Stylistic representation of QT interval prolongation
It should be noted that the landmarks defining the QT interval were deliberately made very clear in Figs. 3.1 and 3.2. In actual cases, the precise identification of these landmarks, particularly the offset of the T-wave, can be extremely difficult to identify, and strategies to optimize the accuracy of identification are discussed in Chap. 7 in the context of proarrhythmic cardiac safety research.
Cardiac cells are surrounded by a membrane, and the cell’s intracellular environment has a negative electrical charge compared with the cell’s immediate extracellular environment. The voltage difference across the cell membrane is termed the transmembrane potential. In between cardiac contractions, the resting transmembrane potential is around minus 80 millivolts (−80 mV) to −90 mV. The greater negativity inside the cell results from an accumulation of relatively more negatively charged ions than positively charged ions inside the cell. Intrinsic electrical stimulation from the heart’s natural pacemaker causes a cascade of ion channels in the cell’s membrane to open and close rapidly. In simple terms, depolarization (which means the transmembrane potential moving back towards zero) is achieved by an influx of positively charged sodium ions through sodium ion channels. This is associated with the initiation of the cardiac contraction or heartbeat. Repolarization (the potential moving back towards −80 mV) is achieved by the efflux of positively charged potassium ions: less positive charges within the cell means that the transmembrane potential moves towards a more (negatively) polarized level again. In more accurate terms, the change in transmembrane potential from the resting level at each point in time across the cardiac cycle is the net result of incoming and outgoing ions moving through multiple sodium and potassium ion channels (and also calcium channels and ion pumps, which for simplicity are not discussed in this chapter) resulting in local changes in net electrical charge on either side of the cell membrane. These resulting voltage fluctuations can be graphed against time to yield the cell’s action potential.
A stylistic representation of the action potential, represented as phase 0 through phase 4, and ionic currents influencing the action potential at each phase can be seen as Fig. 1a in an Open Access paper by Sager and colleagues (2014), and a detailed description of the ionic currents of particular relevance to related discussions in Chap. 9 is presented in Table 9.1. The voltage spike resulting from a given cell’s depolarization tends to cause adjacent cardiac cells to depolarize by causing their sodium channels to open. Once a cardiac cell is stimulated to depolarize, an electrical impulse is propagated across the heart, cell by cell, by a wave of depolarization. The speed of a cell’s depolarization is reflected by the slope of phase 0 of the action potential: the steeper the slope (i.e., the closer it is to vertical), the faster the rate of depolarization.
Once a cardiac cell has been depolarized, it cannot be depolarized again until repolarization has occurred. At normal resting heart rates (around 75 beats per minute for adult males, and somewhat higher for adult females), there is less than one second for both depolarization and repolarization to occur: this timespan decreases considerably at heart rates that are achieved during vigorous exercise. Therefore, repolarization must happen (very) quickly. The time taken by the process of repolarization is called the refractory period. Its length corresponds roughly to the time for phases 1 through 3 of the action potential to occur.
3.3 Cardiovascular System Parameters of Interest
Four cardiovascular parameters of interest are stroke volume, cardiac output (CO), total peripheral resistance of the systemic vasculature (TPR), and blood pressure. Measurements of both systolic blood pressure (SBP) and diastolic blood pressure (DBP) are typically presented when discussing blood pressure.
3.3.1 Stroke Volume and Cardiac Output
Stroke volume is the amount of blood pumped into the aorta per heartbeat. It is typically measured in milliliters (ml), and a typical stroke volume is around 60–100 ml. Cardiac output is the total amount of blood pumped per unit of time, typically 1 min. As is the case for heart rate, CO is usually represented in intervals of 1 min and is measured in liters per minute (lpm). A typical CO for an adult resting quietly is about 5–6 lpm. This can increase several fold as needed during physical activity: it can also increase during emotional stress.
3.3.2 Total Peripheral Resistance of the Systemic Vasculature
Along with CO, TPR is the second primary determinant of blood pressure. The simultaneous measurement of blood pressure and CO allow the derivation of TPR. It is the resistance provided by the vasculature to the ejection of blood into the aorta. Blood pressure can be thought of as representing a manifestation of the interaction between the heart and the vasculature (Obrist 1981). A given change in blood pressure can be the result of a change in cardiac output, a change in TPR, or a combination of both.
3.3.3 Blood Pressure
There is continuous pressure in arteries to propel blood through them. The level of blood pressure fluctuates during each cardiac cycle. Pressure is highest during systole (contraction) when blood is ejected from the left ventricle into the aorta: this pressure is labeled SBP. Pressure is lowest during diastole (relaxation) and labeled DBP.
The unit of blood pressure measurement received its name, millimeters of mercury (mmHg), during a period of time in which a catheter was placed into the brachial artery in the arm, and blood was channeled to the bottom of a tube of mercury. The height of the mercury column varied during the cardiac cycle due to the fluctuations in blood pressure: the pressure at each point in time caused the column of mercury to rise to a certain height. This height was measured in millimeters, and hence the unit mmHg. Modern measurement techniques are noninvasive in nature and no longer require the use of mercury.
Given the importance of blood pressure measurement to diagnose high blood pressure, or hypertension (see Sect. 3.4.4), a methodological incongruity should be highlighted here: while it is remarkably (and arguably scarily) easy to obtain a blood pressure reading, it can be disturbingly difficult to estimate the correct blood pressure reading in a given circumstance (Turner 2010). Going one step further, it is not as straightforward as one might imagine to operationally define “correct” in this context. Pickering and colleagues provided useful insights, observing that “Any clinical measurement of blood pressure may be regarded as a surrogate measure for the ‘true’ blood pressure of the patient, which may be defined as the mean level over prolonged periods” (Pickering et al. 2006). In general medical practice, it is common for a single blood pressure to be taken by a physician or other health-care professional. Two questions therefore arise: Is the reading taken an accurate representation of blood pressure at that moment? And, even if it is an accurate measurement, is it a meaningful representation of the patient’s “true” blood pressure, defined, as just noted, as the mean level over prolonged periods of time?
An alternate blood pressure measurement modality involves multiple readings being taken by a fully automated device while the patient rests quietly and alone in a room within the physician’s office suite. Advantages include improved accuracy, reduced digit preferences (e.g., the tendency of a human reader to “round up” or “round down” values to the nearest value ending in a zero or a five), the reduction of the influence behind white-coat hypertension (discussed momentarily), and a stronger relationship between readings taken in this manner with target organ damage compared with manual readings (Andreadis et al. 2011). Myers and colleagues have advocated for automated office blood pressure measurement to replace traditional office blood pressure assessment (Myers 2010, 2014; Myers and Godwin 2012).
In the last two decades, a considerable literature discussing home blood pressure monitoring has accumulated (see Stergiou et al. 2014). Stergiou and colleagues have discussed advantages of home blood pressure monitoring, including the following: prediction of preclinical target organ damage and cardiovascular events in a manner superior to clinic blood pressure measurements and improvement of long-term adherence to antihypertensive drug treatment in treated hypertensive patients and hence improved hypertension control rates. The authors commented that, as long as current recommendations are followed, home blood pressure monitoring should have a “primary role in diagnosis, treatment adjustment, and long-term follow-up” of most cases of hypertension (Stergiou et al. 2014). It should be noted, however, that home blood pressure gives no indication of blood pressure during sleep (O’Brien et al. 2013).
Ambulatory blood pressure monitoring, widely considered to be the “gold standard” for evaluating true levels of blood pressure over a prolonged period of time, facilitates collection of blood pressure readings several times an hour across a 24-h period (Turner et al. 2015). These readings can be aggregated to yield 24-h means and also grouped into time windows, e.g., mean daytime and nighttime values (Pickering et al. 2006; O’Brien 2012). These various blood pressure categorizations are valuable for clinical management of high blood pressure because they increase the accuracy for diagnosis and the prediction of cardiovascular risk (Krakoff 2013). Ambulatory blood pressure monitoring enables white-coat hypertension to be ruled out, thus precluding patients who do not need pharmacologic interventions at that time from being prescribed such intervention, while also identifying those individuals who should commence antihypertensive therapy. It also facilitates the assessment of BP during sleep time: a non-dipping pattern and nocturnal hypertension are strongly associated with increased cardiovascular morbidity and mortality (Turner et al. 2015).
3.4 Cardiac and Cardiovascular Diseases and Occurrences of Clinical Concern
While there are multiple cardiac and cardiovascular diseases, this section presents brief reviews of a relatively small subset that are of relevance to discussions later in the book.
3.4.1 Arrhythmias
Arrhythmias, abnormal cardiac rhythms, are disorders of cardiac impulse generation and propagation. Both atrial and ventricular arrhythmias can occur. The term bradycardias refers to slower than usual patterns of cardiac contractions: tachycardias are faster than usual patterns.
Atrial arrhythmias include atrial flutter and atrial fibrillation. During atrial flutter, the atria contract in a regular but very rapid pattern. During atrial fibrillation (AFib), the number of beats per minute is even higher, and, of importance, the pattern is irregular. At least 30 million people worldwide carry a diagnosis of AFib, and many more likely have undiagnosed, subclinical, or “silent” AFib. Atrial fibrillation-related cardiovascular morbidity and mortality includes heart failure, stroke, hospitalizations, and cardiovascular deaths (Kirchhof et al. 2016).
Ventricular arrhythmias include premature ventricular contractions, ventricular tachycardia, and ventricular fibrillation. Premature ventricular contractions occur when the ventricles occasionally contract earlier than usual, and the electrical origin of the beat is within the ventricle and not the atria or the atrioventricular node. This can often be felt as a “skipped” heartbeat, a common condition that is typically not of clinical concern. However, two or more of these occurring together can be a warning sign of more serious arrhythmias. Ventricular tachycardia can be caused by irritated myocardial cells, for which there are multiple reasons. These cells cause a regular but rapid heart rate that often leads to inefficient pumping. Ventricular tachycardia can deteriorate to ventricular fibrillation, the major underlying cause of sudden cardiac death (Boukens et al. 2015). This occurs when myocardial cells contract in a totally disorganized fashion, resulting in the heart no longer pumping blood around the body.
3.4.2 Torsades de Pointes
Torsades de pointes (torsades) (Dessertenne 1966; Fabiato and Coumel 1991) is a particular form of ventricular tachycardia that has three defining characteristics on an ECG recording:
It is associated with QT interval prolongation.
The shape of the QRS complex (QRS morphology) twists around an imaginary axis.
The QRS complex takes on many morphologies as it twists around this imaginary axis, leading to the descriptor polymorphic.
The first characteristic listed indicates that the term torsades is reserved for polymorphic ventricular tachycardia associated with QT interval prolongation: in the absence of QT prolongation, the descriptor should simply be polymorphic ventricular tachycardia.
3.4.3 Cardiac Channelopathies I: Long QT Syndrome
Inherited ion channel pathophysiologies are referred to as channelopathies. Some cardiac channelopathies lead to inherited long QT syndrome (LQTS) (see Nakano and Shimizu 2016). Bunch and Ackerman (2007) observed that the discipline of cardiac channelopathies began in 1995 with the discovery of mutations in genes encoding critical cardiac ion channels. Schwartz (2005, p.186) commented on this occurrence as follows:
The identification at the end of March 1995 (Wang et al. 1995; Curran et al. 1995) of the first two long QT syndrome (LQTS) genes represented a major breakthrough not only for cardiac electrophysiology but also for cardiology as a whole and paved the way for the understanding of how tight the relationship between molecular and clinical cardiology can be. Indeed, the impressive correlation between specific mutations and critical alterations in the ionic control of ventricular repolarization has made LQTS the best example to date for the specificity and value of the correlation between genotype and phenotype.
There are multiple LQTSs, each of which is the result of a specific genetic mutation. Given our primary focus on potassium and sodium cardiac ion channels (calcium channels are addressed in Chap. 9), Table 3.1 presents three LQTSs as examples: LQT1, LQT2, and LQT3. In each case, the gene in which the mutation occurs, the ionic current that is affected, and the consequence of the genetic mutation are listed. These mutations involve either loss-of-function potassium ion channel mutations (less current flows through the channel) or gain-of-function sodium ion channel mutations (more current flows through the channel, or current flows for a longer period of time).
Table 3.1
Long QT syndromes LQT1, LQT2, and LQT3
Channelopathy | Gene in which mutation occurs | Ionic current affected | Consequence of mutation |
---|---|---|---|
LQT1 | KCNQ1 | I Ks | Decrease in the repolarizing I Ks potassium current: net repolarizing influence is lessened |
LQT2 | KCNH2 | I Kr | Decrease in the repolarizing I Kr potassium current during repolarization: net repolarizing influence is lessened |
LQT3 | SCN5A | I Na | Gain of function in (or persistence of) the I Na sodium current, a depolarizing influence: net repolarizing influence is lessened |
LQTS may be detected via the identification of QT prolongation on an individual’s ECG before any clinical manifestations present themselves. However, given the complexities of identifying QT prolongation in many cases, a topic discussed in detail in Chap. 7, such detection does not always happen. Clinical manifestations of LQTS can be dramatic and very dangerous: it can present as abrupt-onset syncope (loss of consciousness), seizures, or sudden cardiac death due to torsades. Some sudden deaths having origins in a channelopathy may have been presaged by unrecognized warning signs including syncope brought on by physical exertion or by a positive family history of premature sudden death where causes were not identified.
Detailed discussions of drug-induced QT interval prolongation later in the book make LQT2 of particular relevance here. As seen in Table 3.1, this LQTS results from a decrease in I Kr. For reasons of molecular biology and the interaction of small-molecule drugs with biological structures, it happens to be the case that drug-induced QT prolongation is commonly produced as the result of drug molecules being trapped inside the cardiac channel through which I Kr flows: in other words, it is the result of a decrease in I Kr. Given that LQT2 is of considerable concern and that drug-induced QT prolongation is commonly the result of a decrease in the same ionic current as is seen in LQT2, drug-induced QT prolongation also becomes of clinical concern.
It is worth noting here that while discussion in subsequent chapters rightly focuses on the unwanted consequences of drug-induced QT prolongation, the identification of nonlethal drug-induced QT prolongation in an individual can sometimes be the sentinel event leading to the serendipitous identification of the inherited disorder LQTS: the individual may then benefit from clinical intervention (Turner and Durham 2009).
Section 3.5 reviews a different set of channelopathies comprising cases of short QT syndrome (SQTS). While these channelopathies and their drug-induced phenotypic counterparts are not of central importance in this book’s discussions, they are certainly worthy of inclusion in this chapter.
3.4.4 Hypertension
High blood pressure is currently the greatest threat to the global burden of disease (Horton 2013; Das and Samarasekera 2013; Lim et al. 2013): it continues to be the most common diagnosis in adult primary care practice and the most salient risk factor for cardiovascular disease (Turner et al. 2015). Hypertension is the most common cardiovascular disease in the USA and many other industrialized countries: for example, approximately 80 million adults in the USA have been diagnosed with high blood pressure (American Heart Association web site). Two characteristics make hypertension particularly dangerous. First, it has no direct symptoms: an individual can have high blood pressure for years without being aware of this condition (hypertension is often discovered while visiting the doctor for another complaint). Second, it contributes strongly to the etiology of several other cardiovascular diseases. Taken in conjunction, these characteristics mean that considerable arterial and organ damage can occur during the time that hypertension remains undetected.
The American Heart Association’s (AHA’s) web site presents several blood pressure categories, shown in Table 3.2:
Table 3.2
Blood pressure categories defined by the AHA
Category | SBP (mmHg) | DBP (mmHg) | |
---|---|---|---|
Normal | <120 | And | <80 |
Prehypertension | 120–139 | Or | 80–89 |
Stage 1 hypertension | 140–159 | Or | 90–99 |
Stage 2 hypertension | 160 or higher | Or | 100 or higher |
Hypertensive crisis (emergency care is needed) | >180 | Or | >110 |
3.4.5 Myocardial Infarction
Approximately 500,000 episodes of acute myocardial infarction occur each year in the USA (Wang et al. 2015). The area of myocardium damaged by a heart attack depends on which coronary artery was blocked, preventing blood and hence oxygen from reaching that area.
The precise definition of a myocardial infarction is a subject of detailed discussion in the literature. Different definitions can be used in clinical practice, clinical trials, and registries (White et al. 2014). In an attempt to standardize definitions, the Third Universal Definition of myocardial infarction is based on troponin elevation together with ischemic symptoms, ischemic ECG changes, and imaging evidence: myocardial infarctions are classified into several types according to whether they are spontaneous, secondary to imbalance between coronary artery blood supply and demand, related to sudden death, or related to revascularization procedures (White et al. 2014).
The typical pain from a heart attack may feel like angina at first but usually becomes more widespread and more severe and lasts longer. Heart attack victims often experience weakness, sweating, and a fear of dying in addition to their chest pain. However, if only a small area of myocardium is injured, it may cause relatively little chest pain that may be mistaken for heartburn. Some patients can have a small heart attack without experiencing any pain. Patients who survive a heart attack are at risk for developing several complications, including arrhythmias and heart failure.
3.4.6 Heart Failure
The term heart failure should not be taken in the literal sense that the heart has failed and is not pumping any blood and death therefore ensues in a matter of minutes if no action is taken. Rather, heart failure refers to a condition where the heart is pumping in a less-than-optimal manner (AHA web site). The heart is therefore providing a less-than-optimal oxygen supply, resulting in shortness of breath and fatigue during everyday activities.
Heart failure is a major public health concern (Gedela et al. 2015). It has been recently categorized into heart failure with reduced and preserved ejection fraction: mortality remains similarly high for both conditions.
The heart can implement various compensatory strategies. The chambers of the heart can enlarge (dilatation), or the heart muscle can thicken (hypertrophy) depending on the etiology of the problem. Usually both will occur which allows the heart to maintain cardiac output, at least at first. If hypertrophy alone occurs, generally the amount of blood pumped with each beat will be reduced. The undesirable consequences of heart enlargement include bodily fluid retention affecting the lungs (the term congestive heart failure is sometimes seen) and arrhythmias. As the condition progresses, the body will divert blood away from certain tissues it considers less essential, such as the kidneys and skeletal muscle, to preserve flow to the brain.
3.5 Cardiac Channelopathies II: Short QT Syndrome
As Antzelevitch and Francis (2004) noted, inherited LQTS was first described in individuals with structurally normal hearts six decades ago by Jervell and Lange-Nielsen (1957). In contrast, inherited SQTS was first proposed as a new inherited clinical syndrome less than two decades ago by Gussak and colleagues (2000). Included in their clinical report was a description of three members of one family: a 17-year-old female, her 21-year-old brother, and their 51-year-old mother. The sister’s QT interval was 280 msec at a heart rate of 69, the brother’s QT interval was 272 msec at a heart rate of 58, and the mother’s QT interval was 260 msec at a heart rate of 74. The shorter than usual QT interval was associated in the sister with several episodes of paroxysmal atrial fibrillation requiring electrical cardioversion. Similar ECG changes seen in a 37-year-old individual not related to the family were associated with sudden cardiac death (Gussak et al. 2000).
Another report of interest was provided by Gaita and colleagues (2003). Several members of two different families were referred for syncope, palpitations, and resuscitated cardiac arrest in the presence of a positive family history for sudden cardiac death. All individuals displayed no evidence of structural heart disease and had a constantly and uniformly short QT interval: at baseline, they all exhibited a QT interval ≤ 280 ms and QTc ≤ 300 ms. The authors concluded as follows (Gaita et al. 2003):
The short QT syndrome is characterized by familial sudden death, short refractory periods, and inducible ventricular fibrillation. It is important to recognize this ECG pattern because it is related to a high risk of sudden death in young, otherwise healthy subjects.
A year later, Brugada and colleagues (2004) reported their study of three families with SQTS and a high incidence of ventricular arrhythmias and sudden cardiac death. In two families, a mutation was identified in the hERG gene. As shown in Table 3.1, this is the gene that encodes the hERG channel through which I Kr flows. The mutation in that case, which led to a decrease in the repolarizing I Kr and hence a decreased net repolarizing influence, led to LQT2. In this case, a different mutation led to an increase in I Kr, an increase in net repolarizing influence, and hence a shortened QT interval. As for LQTS, there are multiple forms of SQTS. The form just discussed, a gain of function in the hERG channel and associated QT shortening, is called SQT1.
3.5.1 Origins of Regulatory Interest in Drug-Induced QT Interval Shortening
As discussed in detail in Chap. 7, realization that some drugs could lead to the same phenotype as LQTS (a prolonged QT interval), led to scientific, clinical, and regulatory interest in this phenomenon in the late 1990s. This in turn led to the release in 2005 of two guidelines from the International Council for Harmonisation of Technical Requirements for Pharmaceuticals for Human Use (ICH), which have subsequently been adopted by regulatory agencies. ICH S7B focused on nonclinical examination of drug-induced decreases in I Kr. ICH E14 focused on preapproval clinical assessments of drug-induced prolongation.
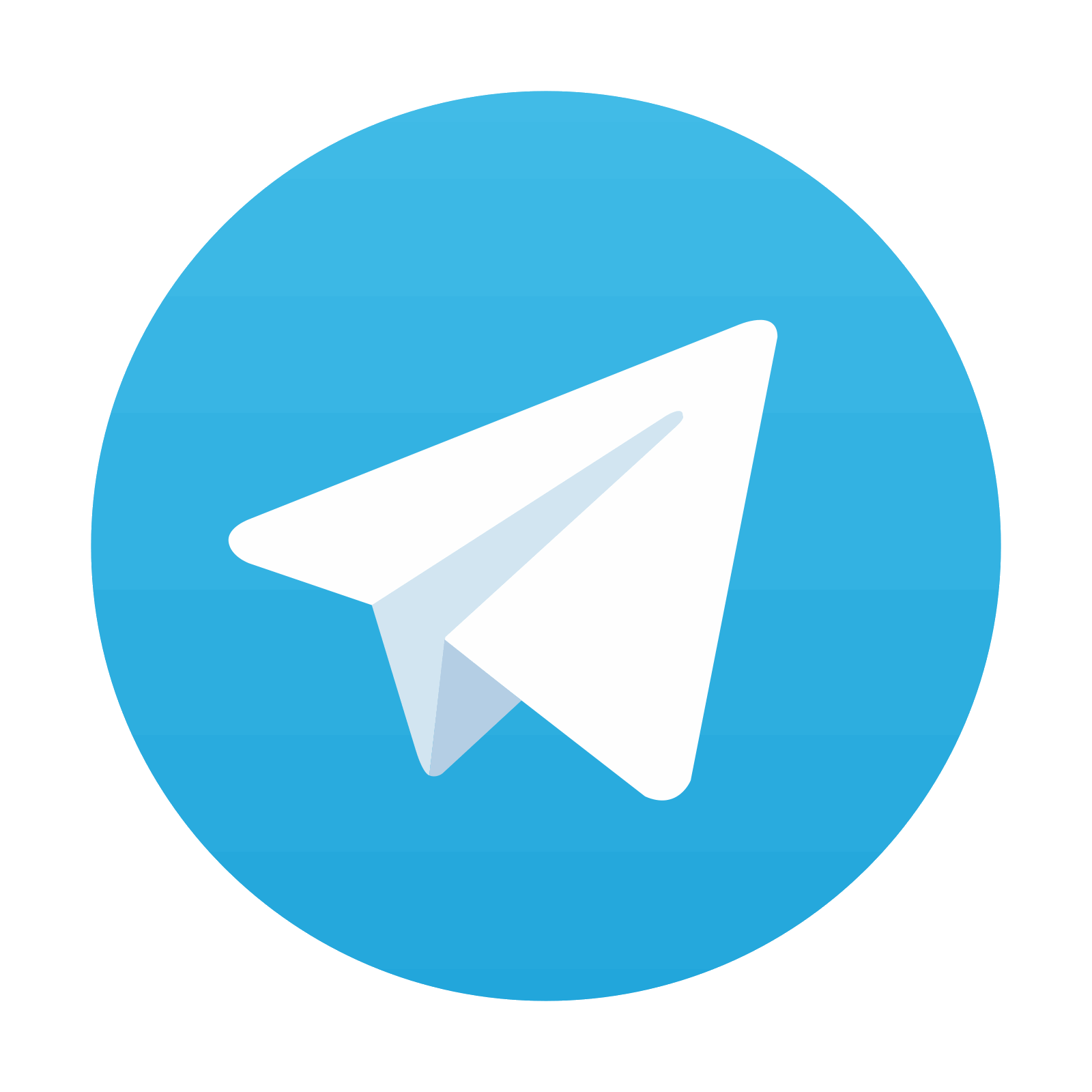
Stay updated, free articles. Join our Telegram channel
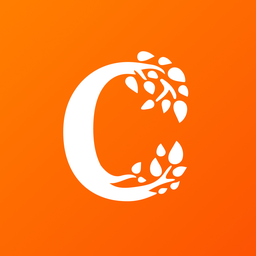
Full access? Get Clinical Tree
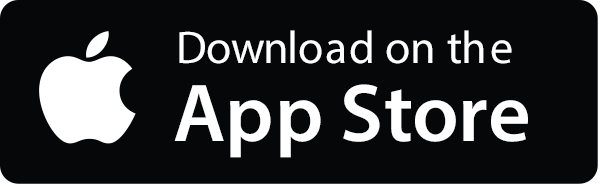
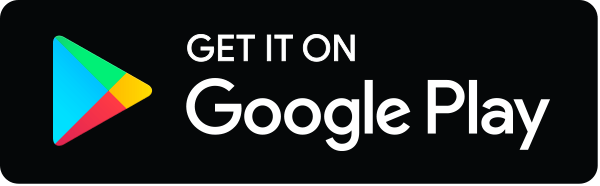