Fig. 17.1
Example vascular grafts. a 300 mm diameter aortic graft made from woven polyester. The graft has a crimped structure which helps to prevent collapse of the lumen. Both external and internal surfaces are textured (velour) which enhances integration into the surrounding tissues and promotes the adhesion and growth of endothelium on the luminal surface. b 6 mm expanded PTFE graft for femoral artery bypass
17.2.2 Complications and Failure Modes
Large diameter grafts are generally regarded as successful but problems arise with medium and small diameter prostheses. This is a significant issue since vessels of the leg (e.g. femoral artery circa 6 mm diameter) and the coronary arteries (circa 3 mm diameter) are commonly affected by atheroma. Thrombosis is reported to be a complication in the early post-operative period but, in the medium- to long-term there is growth of a fibrous lining within the graft. This is called neointimal hyperplasia (NH) and is due to proliferation and migration of smooth muscle cells and increased production of extracellular matrix. NH can lead to a significant reduction of lumen diameter and loss of patency (becoming obstructed). Compliance mismatch between the natural vessel and the graft is believed to play a role due to both mechanical strain and wall shear stress (WSS) effects as described by Salacinski et al. (2001). The sensitivity of the natural vessel to variations in WSS is discussed in Chap. 5. The most commonly used graft materials are, in general, stiffer than the natural vessel. In addition most grafts are secured to the host vessel using sutures. This damages the natural vessel wall and also introduces local stiffening. Computational fluid dynamic (CFD) studies of typical graft/vessel geometries have shown that the anastomotic sites (the sites of surgical joining of the two vessels) can result in altered WSS. Natural pulsatile changes in arterial diameter will also affect the WSS close to the stiffer anastomosis. Whilst, as discussed in Chap. 5, arteries respond to changes in WSS by a change in diameter, the diameter of the graft remains unchanged.
17.3 Vascular Stents
A vascular stent is an expandable tube which is inserted into an artery to correct a narrowing or support a weakness in the vessel wall. Stents are commonly used to improve flow in diseased coronary arteries but they are also used in the carotid, iliac, and femoral arteries. Stents can also be used in conjunction with a fabric graft (stented graft) which can be placed within the aorta, for example, to provide a strong repair for an aneurysm or, as will be described later, to introduce a heart valve into the aorta.
Here, we will focus on coronary stents. In the treatment of coronary artery stenosis, stenting provides a less invasive alternative to bypass surgery using a graft.
17.3.1 Balloon Angioplasty and Stent Deployment
Percutaneous Transluminal Angioplasty (PCTA) was first introduced in 1977 (Meier et al. 2003). This procedure involves the introduction of a catheter, via a thin guidewire, into the radial or femoral artery. The guidewire is advanced through the circulation to the coronary arteries under angiographic guidance with the aid of a radio-opaque contrast medium allowing the catheter to be positioned at the site of the occlusion. The end of the catheter carries a collapsed non-compliant balloon. This is aligned with the plaque and inflated with saline and contrast medium using a hand pump to carefully apply the pressure. The balloon displaces the plaque and restores the vessel lumen.
There are two major drawbacks to what at first appears to be a relatively simple process. First, there is a risk of immediate elastic recoil of the vessel wall when the balloon is removed resulting in narrowing the lumen. Second, injury to the vessel wall caused by balloon inflation may lead to an adverse biological response involving the proliferation and migration of smooth muscle cells from the vessel media into the lumen and the deposition of extracellular matrix. This remodelling process is known as neointimal hyperplasia (NH). The magnitude of the biological response has been shown to be related to the degree of injury and may result in the gradual restenosis of the diseased vessel. Restenosis affects an estimated 40 % of cases within 3–6 months of ballooning (Dangas and Kuepper 2002).
Elastic recoil can be prevented by introducing a stent into the vessel via the balloon. Expansion of the stent (stent deployment) provides a scaffold to support the vessel wall maintaining patency. The first balloon-expandable stents were proposed by Palmaz et al. in (1985) and a range of different designs have been used in clinical practice since the 1990s and are now used by cardiologists across the world. More detail is available in a review by Iqbal et al. (2013). A catheter, balloon with stent and pump are shown in Fig. 17.2 and the balloon and stent are shown in greater detail in Figs. 17.3 and 17.4.




Fig. 17.2
PCTA system for the delivery of a coronary artery stent comprising; balloon-tipped catheter with non-compliant balloon and mounted stent and a hand pump for inflating the balloon. The same system without the stent can be used for PCTA alone

Fig. 17.3
Balloon and mounted stent are shown in greater detail prior to inflation

Fig. 17.4
Detail of the inflated balloon and expanded stent
An ideal stent should be flexible and have good radiographic trackability and radial strength, have low radial and longitudinal recoil and be able to be expanded symmetrically (reducing the likelihood of high local wall strain and deep injury to the vessel wall with local penetration of the stent struts).
Whilst NH remains the primary complication, stenting has been shown to halve the number of cases experiencing restenosis (from 40 % reported with ballooning alone to 25 %, Serruys et al. 1994). NH formation has been shown to be related to changes in stress and strain distribution in the artery wall due to the force of the deployed stent. Thicker struts offer better support to the arterial wall but are associated with increased neointimal response. This is thought to be due to the greater degree of injury imposed (Zahedmanesh and Lally 2001). There is a clear link between the magnitude of the NH response and the magnitiude of stresses and strains during stent deployment as shown by Gunn et al. (2002).
17.3.2 Types of Stent
The first stents were manufactured from 316L stainless steel. 316L Stainless steel has the benefit of acceptable material properties with adequate corrosion-resistance and good biocompatibility but it has some limitations in terms of the thickness of strut needed to ensure adequate radio-opacity, and radial strength which can have a negative effect on the flexibility of the overall device. Recent designs use cobalt chromium alloy or, more recently still, platinum chromium alloy (Jorge and Dubois 2015), giving the required radial strength, improved flexibility and radio-opacity in designs with thinner struts. Despite the known risk of NH and restenosis, bare–metal stents (BES) are used in 15–20 % of procedures (Alfonso et al. 2014).
Drug–eluting stents (DES) offer a potential solution to the problem of NH. Restenosis can be significantly reduced or even prevented by local controlled release of an anti-proliferative drug. The drug is incorporated into a thin polymer carrier material coated onto a bare-metal stent (Nabil and Braunwald 2012).
Although clinical experience with DES indicates clear benefits in terms of reduced incidence of restenosis, it also reveals drawbacks. The mechanical impact of deploying the stent partially or completely removes the protective anti-thrombotic layer of endothelium in the vicinity of the stent. Reports show that, whilst mitigating against NH, the anti-proliferative drug also prevents re-endothelialisation of the stented segment. This can lead to late thrombosis (McFadden et al. 2004). Clinical guidelines recommend antiplatelet therapy is administered for 1 month after using a bare-metal stent but a period of more than 6 months is recommended for DES.
In addition to balloon-expandable stents self-expanding stents are also available. These are constructed from shape memory alloy, typically nitinol. Nitinol, an alloy of nickel and titanium, has unique properties; it has the ability to undergo deformation at one temperature and then return to its original, un-deformed shape when heated above its “transformation temperature”. This enables a stent to be introduced into the artery in its compact shape, constrained by a sheath. Removal of the sheath enables the stent to spring back to its expanded shape. The force generated by the expansion is sufficient to restore the lumen in a blocked vessel. More information on the properties of nitinol and the design of self-expanding stents can be found in Stoeckel et al. (2004).
17.3.3 The Future
Work is already under way to develop a fully resorbable stent. These are intended to perform the same mechanical scaffolding function of a traditional metal stent but for a controlled period of time. This offers the possibility that the natural vasomotor function of the vessel can be re-established (Tamburino et al. 2015). Polymers such as polylactic acid (this has the advantage of breaking down into a naturally occurring molecule—lactic acid) and metals such as magnesium are under consideration. Other novel target areas under consideration are methods to accelerate re-endothelialisation of the stented segment using growth factors or cells delivered on the stent.
17.4 Heart Valves
The heart contains four major valves. These maintain the flow of blood in the forward direction, opening and closing passively in response to pressure changes generated by cardiac contraction. Two valves (aortic and pulmonary) are located at the outflow of the major arteries (aorta and pulmonary trunk) leaving the left and right ventricles, respectively. The aortic and pulmonary valves are semi-lunar valves; they have three, thin, flexible, crescent-shaped leaflets which are strengthened by strong collagen cords. Two further valves (right and left atrioventricular (AV) valves) are located between the upper and lower chambers of the heart. These have a more complex structure; the leaflets are ‘flap-like’. Strong cords of collagen spanning between the free edges of each leaflet and the walls of the ventricles act as ‘guy-lines’ tethering the leaflets and preventing them from turning inside out under the back pressure which is applied as the ventricle contracts.
17.4.1 Disease of Natural Valves
In the open position, healthy valves provide minimal resistance to forward flow. Closure is also very effective with little backflow or leakage. In contrast, diseased valves can result in major haemodynamic disturbances which cause increased workload for the heart. Valve disease has a number of potential underlying causes including congenital defects (present at birth), infection or age-related degeneration. More than 10 % of the population aged 70 or over have diseased valves. An introduction to valve disease and current treatment guidelines can be found in Morris et al. (2015). Whilst all valves in the heart can be affected by disease, in reality those in the left side of the heart (the aortic and left AV valves) are most frequently affected. This is related to the greater load experienced due to the pressure regime in the systemic circulation.
Valve disease can be categorised as;
Stenotic—leaflets are stiff, restricting the open orifice and presenting increased resistance to forward flow. More energy must be expended by the heart to maintain forward flow.
Regurgitant—the leaflets fail to close or leak allowing regurgitation (backflow of blood) as blood is able to flow back upstream the heart must work harder to maintain the same level of cardiac output.
A combination of stenosis and regurgitation is also possible.
The pressure difference (pressure gradient) across the open valve provides a measure of stenosis whilst the regurgitant fraction (percentage of the stroke volume leaking back through the valve) provides a measure of leakage. Quantitative evaluation of valve haemodynamics can be made clinically using trans-oesophageal echocardiography (TOE-Chap. 9). Haemodynamic diagnostic markers of severe disease include a stenotic valve area <1 cm2 or a regurgitation fraction of >50 %. Ultimately, for the individual, the physiological impact of valve failure will depend on the ability of the heart to adapt to the increasing workload. If the physiological impact becomes severe the diseased valve can be replaced by an artificial valve.
There are a number of requirements for a successful replacement valve. First and foremost, it must have adequate durability, i.e. withstand opening and closing 40 million times a year, ideally for the life-time of the patient. It must also have good haemodynamic characteristics including; a large orifice area to offer minimal resistance to forward flow, allow minimal backflow, create minimal flow disturbance and not cause regions of high shear stress (which could lead to blood cell damage, see Chap. 3). In addition, the materials used must be non-thrombogenic (i.e. not cause activation of the blood).
17.4.2 Types of Artificial Heart Valves
Two different types of replacement valves are available; mechanical valves which are made from man-made materials and biological (tissue) valves which are made from a combination of synthetic materials and chemically stabilised collagen-based animal tissues. A support structure and a fabric cuff, enabling the valve to be sutured in place within the heart are common features of both types of valve. More information on the range of designs and their evolution can be found in Gott et al. (2003).
Mechanical valves have one, or more commonly 2, moving components (occluder(s)) trapped within a rigid metal or pyrolytic carbon frame (housing), these are free to rotate in response to the changes in pressure field as the heart contracts and relaxes. The components of many of the current generation of mechanical valves are made from pyrolytic carbon, a material that is extremely durable and, importantly, does not cause blood clotting.
Biological valves are made from animal tissue supported on a frame (stented valves) or mounted in a conduit of synthetic fabric or fixed tissue (stentless valves). The tissue component has 3 flexible leaflets (formed from a natural pig aortic valve or fashioned from bovine pericardium, the sac of fibrous tissue that surrounds the heart). These tissues need to be treated with a chemical fixative agent to enhance their mechanical and biological durability. Issues relating to durability are discussed in more detail by Hofmans et al. (2008). Examples of valve models of different designs are shown in Fig. 17.5.


Fig. 17.5
Four different valve designs in the open configuration. a Caged ball mechanical valve with silicone ball and metal housing. b Titling disc valve with single pyrolytic carbon occluder captured in a metal housing. c Bileafet valve with two semi-circular pivoting pyrolytic carbon occulders. d Bioprosthetic valve, chemically stabilised porcine valve mounted on a fabric covered frame
Valve replacement, although now routine, is a major undertaking as it involves opening the heart; the damaged valve is excised and a suitable artificial valve is sutured in its place. The specific valve type is chosen based on factors such as patient age, and the ability to comply with anticoagulation treatment. Tissue valves are susceptible to calcification in the young, but in adults the expected durability of the most recent designs is in excess of 12 years. Mechanical valves are associated with haemodynamic disturbances which may increase the risk of thromboembolism.
Concerns about the high risks associated with surgery have driven the development of percutaneous (meaning ‘through the skin’) valve implantation. This more recently introduced procedure (currently only for aortic replacement) is also known as trans-catheter aortic valve implantation (TAVI). A review of TAVI and the current state of the art is provided by Collas et al. (2014). In TAVI valves tissue leaflets are mounted on an expandable wire stent and delivered into the aorta on a catheter. A number of different approaches can be used including introduction via the femoral artery in the thigh to the site of the damaged aortic valve. This procedure is guided by fluoroscopy (Chap. 9). Stents are either balloon-expandable or self-expanding, and once correctly located, the stent is expanded by inflating a balloon on a catheter within the orifice or by removing a constraining sheath releasing the self-expanding stent.
17.4.3 Valve Performance
All replacement valves present some degree of obstruction to flow. From Fig. 17.6 which illustrates three different occluder configurations of mechanical valves, it can be seen that the early ball and cage design, first introduced in the 1960s, is much more obstructive than the tilting disc and bileaflet designs which are in current use. Whilst tissue valves should present less obstruction due to the central flow, the supporting frame and cover adds bulk to the design, limiting the size of the valve that can be implanted. For this reason, stentless valves have been introduced. Stentless valves lack a stiff supporting frame which means that they can only be implanted in the aortic position.
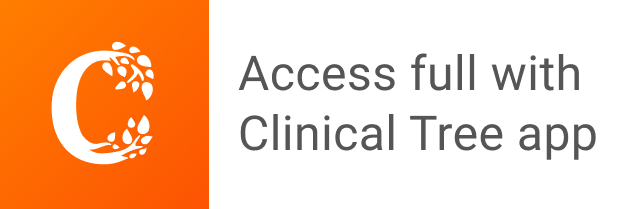