Cardiovascular pathology defines the morphology and mechanisms of cardiovascular disease in individual patients and patient cohorts, both along the natural history of a disease and following surgery and interventions. The data derived through cardiovascular pathology thereby facilitate evidence-based choices among surgical or catheter-based interventional options and optimize short- and long-term patient management. Beyond implicit clinical benefit for individual patients, the discipline of cardiovascular pathology is a cornerstone of modern cardiovascular research and the preclinical development and clinical implementation of innovative drugs, devices, and other therapeutic options.
This chapter summarizes pathologic anatomy, clinicopathologic correlations, and pathophysiologic mechanisms in the various forms of structural heart disease most relevant to surgery and catheter-based interventions used to diagnose and treat the major forms of acquired structural cardiovascular disease. In view of space limitations, several important areas (eg, aortic disease) are necessarily omitted from discussion and others (eg, cardiac assist and replacement devices) are focused on details not covered elsewhere in this book. Moreover, although we have not included the key considerations herein, we are mindful that the number of adults with congenital heart disease is increasing rapidly and that they have unique and important clinical and pathologic concerns.1,2
Hypertrophy is the compensatory response of the cardiac muscle (the myocardium), to increased work (Fig. 5-1).3 This structural and functional adaptation accompanies nearly all forms of heart disease, and its consequences often dominate the clinical picture. Hypertrophy induces an increase in the overall mass and size of the heart that reflects an increased size of individual myocytes largely through addition of contractile elements (the sarcomeres) and associated cell and tissue elements. Substantial and functionally beneficial augmentation of myocyte number (hyperplasia) in response to stress or injury has not been demonstrated in the adult heart.
FIGURE 5-1
Summary of the gross and microscopic changes in cardiac hypertrophy. (A) Gross photo of heart with hypertrophy caused by aortic stenosis. The wall of the left ventricle is thick and the chamber is not dilated. The left ventricle is on the lower right in this apical four-chamber view of the heart. (B) Altered cardiac configuration in left ventricular hypertrophy without and with dilation, viewed in transverse heart sections. Compared with a normal heart (center of this panel), a pressure overloaded heart, caused for example by aortic valve stenosis (left), has increased mass and a thick left ventricular wall, whereas a volume overloaded heart, caused for example by mitral valve regurgitation, is both hypertrophied and dilated (right), having increased mass with a near normal or diminished wall thickness. (C) Photomicrograph of normal myocardium. (D) Photomicrograph of hypertrophied myocardium at same magnification as (C), showing large cells with enlarged. ([B] Reproduced with permission from Allen HD, Gutgesell HP, Clark EB, et al: Moss and Adams’ Heart Disease in Infants, Children, and Adolescents: Including the Fetus and Young Adults. 6th ed. Philadelphia: Lippincott Williams & Wilkins; 2001. [C and D] Reproduced with permission from Kumar V, Fausto N, Abbas A, et al: Robbins/Cotran Pathologic Basis of Disease, 8th ed. Philadelphia, WB Saunders, 2010.)




The pattern of hypertrophy reflects the nature of the stimulus (see Fig. 5-1B). Pressure overload (eg, in systemic hypertension or aortic stenosis) induces an increased ventricular mass, increased wall thickness, and increased ratio of wall thickness to cavity radius without dilation. In contrast, volume overload (eg, in aortic or mitral regurgitation, myocardial infarction, or dilated cardiomyopathy) promotes hypertrophy accompanied by chamber dilation, in which both ventricular radius and total mass are increased. The chamber wall is affected globally by the increased chamber pressure of hypertension, the pressure or volume overload of valvular heart disease, and in dilated cardiomyopathy. In contrast, the ischemic myocyte necrosis and loss of contractile tissue myocardial infarction induce hypertrophy only in noninfarcted regions of myocardium. The terms concentric, eccentric, and compensatory have previously been used to describe pressure, volume, and ischemic injury-related hypertrophy, respectively.
The constellation of changes that occur regionally following myocardial infarction, or more globally in pressure and volume overload, is called ventricular remodeling.4 At a cell level, pressure overload promotes augmentation of cell width via parallel addition of sarcomeres; in contrast, volume overload and/or dilation stimulate augmentation of both cell width and length via both parallel and series addition of sarcomeres.
Hypertrophic changes initially increase the efficiency of the heart, enhance function, and are thereby adaptive. However, when these changes are excessive and prolonged, they may ultimately become deleterious and contribute to cardiac failure via several mechanisms. Because the vasculature does not proliferate and blood flow is not augmented commensurate with increased cardiac mass, hypertrophied myocardium is vulnerable to ischemic damage. Moreover, myocardial fibrous tissue is often increased. Also important are the molecular changes that accompany and likely mediate enhanced function in hypertrophied hearts, and which may subsequently promote development of heart failure. For example, hypertrophy induces a gene expression profile in cardiac myocytes that is similar to that of proliferating cells generally and particularly that of fetal cardiac myocytes during development. Differentially expressed proteins may be less functional and/or more or less abundant than normal. Hypertrophied and/or failing myocardium also may have a mechanical disadvantage engendered by altered chamber configuration, impaired energetics, reduced adrenergic responsiveness, decreased calcium availability, impaired mitochondrial function, microcirculatory spasm, and apoptosis of cardiac myocytes. Novel therapeutic strategies for heart failure treatment based on molecular mechanisms are under investigation.5
Owing to the totality of the changes described above, cardiac hypertrophy comprises a tenuous balance. The adaptive changes may be overwhelmed by potentially deleterious quantitative or qualitative alterations in structure, function, and biochemistry/gene expression, including cardiac configuration, metabolic requirements of an enlarged muscle mass, protein synthesis, decreased capillary/myocyte ratio, fibrosis, microvascular spasm, cell loss, and impaired contractile mechanisms. Hypertrophy also decreases myocardial compliance and may thereby hinder diastolic filling. In addition, hypertrophy is an independent risk factor for cardiac mortality and morbidity, especially sudden death.6
Heart failure can occur with pressure or volume overload of many causes, owing to both regional and global lesions (Fig. 5-2). Although left ventricular hypertrophy (as measured by left ventricular size and mass) regresses in many cases following removal of the stimulus, the extent of resolution in an individual is unpredictable, and the processes by which recovery of the hypertrophied or failing heart (often termed reverse remodeling occur) are uncertain.7 Moreover, progressive cardiac failure may ensue following cardiac surgery and despite revascularization or hemodynamic adjustment, by structural repair (see Fig. 5-2B). In addition, the markedly increased cardiac muscle mass in a hypertrophied heart may compromise intraoperative myocardial preservation.
FIGURE 5-2
Cardiac failure necessitating heart transplantation. (A) Ischemic heart disease, with a large anteroapical-septal myocardial infarct (with mural thrombus) noted to the left of center of the photo (arrow). (B) Four years following mitral valve replacement with a porcine bioprosthesis for congenital deformity causing mitral regurgitation. ([A] Reproduced with permission from Schoen FJ: Interventional and Surgical Cardiovascular Pathology: Clinical Correlations and Basic Principles. Philadelphia: Saunders; 1989.)


Cardiomyopathies are diseases in which the primary cardiovascular abnormality is in the myocardium. A primary cardiomyopathy comprises a condition solely or predominantly confined to heart muscle, whereas a secondary cardiomyopathy (often called specific heart muscle disease) implies myocardial involvement as a feature of a generalized systemic or multisystem disorder, such as amyloidosis and hemochromatosis, other infiltrative and storage diseases, drug and other toxic reactions, sarcoidosis, various autoimmune and collagen vascular diseases, or neuromuscular/neurologic disorders (eg, Duchenne-Becker muscular dystrophy). Genetic causes of primary cardiomyopathy include hypertrophic cardiomyopathy (HCM), arrhythmogenic right ventricular cardiomyopathy (ARVC), left ventricular noncompaction, the ion channel disorders (eg, long QT and Brugada syndromes), and some cases of dilated cardiomyopathy.8 Acquired causes include myocarditis (inflammatory cardiomyopathy), and stress-provoked (takotsubo), tachycardia-induced, and peripartum cardiomyopathy. Although ischemic heart disease, valvular heart disease, and hypertensive heart disease can lead to a clinical practice resembling dilated cardiomyopathy, the terms ischemic cardiomyopathy, valvular cardiomyopathy, and hypertensive cardiomyopathy are discouraged because these conditions more likely reflect compensatory and remodeling changes induced by another cardiovascular abnormality. In contrast to the atherosclerotic coronary arterial disease that underlies most cases of ischemic heart disease, the epicardial coronary arteries are usually free of significant obstructions in patients with a cardiomyopathy.
In some cases (eg, myocarditis, sarcoidosis, amyloidosis, and hemochromatosis) the cause of a cardiomyopathy may be revealed by light and/or electron microscopic examination of a sample of myocardium obtained by endomyocardial biopsy, and other conditions such as ARVC and HCM have characteristic gross and microscopic features demonstrated at the time of transplantation or autopsy (but not generally by endomyocardial biopsy). In endomyocardial biopsy, also used in the management of the ongoing surveillance of cardiac transplant recipients,9 a bioptome is inserted into either the right internal jugular or femoral vein and advanced under fluoroscopic or echocardiographic guidance through the tricuspid valve, to the apical aspect of the right side of the ventricular septum, yielding 1- to 3-mm fragments of myocardium.
The common variants of cardiomyopathy are illustrated in Fig. 5-3.
FIGURE 5-3
Cardiomyopathies. (A and B) Dilated cardiomyopathy. (A) Gross photo showing four-chamber dilation and hypertrophy. (B) Photomicrograph of myocardium in dilated cardiomyopathy, demonstrating irregular hypertrophy and interstitial fibrosis. (C-F) Hypertrophic cardiomyopathy. (C) Gross photo, showing septal muscle bulging into the left ventricular outflow tract. In the gross photo shown in (D) the anterior mitral leaflet has been moved away from the septum to reveal a fibrous endocardial plaque, caused by systolic anterior motion (see text). In (A) and (C), the LV is on the right side of the photo; in (D) the LV is on the left. (E) Gross photo of left ventricular outflow tract of patient with extensive fibrosis owing to remote surgical septal myotomy/myectomy. (F) Photomicrograph of myocardium in hypertrophic cardiomyopathy demonstrating myofiber disarray, with marked hypertrophy, abnormal branching of myocytes, and interstitial fibrosis. (G and H) Arrhythmogenic right ventricular cardiomyopathy. (G) Gross photograph, showing dilation of the right ventricle (on the right) and near transmural replacement of the right ventricular free-wall myocardium by fat and fibrosis. (H) Photomicrograph of the right ventricular free wall in arrythmogenic right ventricular cardiomyopathy, demonstrating focal transmural replacement of myocardium by fibrosis and fat. Fibrosis (collagen) is blue in the Masson trichrome stain in parts (B), (F), and (H).








Dilated cardiomyopathy is characterized by cardiomegaly usually two to three times of normal weight, and four-chamber dilation (see Fig. 5-3A). The primary functional abnormality in dilated cardiomyopathy is impairment of left ventricular systolic function. Mural thrombi are sometimes present predominantly in the left ventricle, and are a potential source of thromboemboli. The histologic changes in dilated cardiomyopathy, comprising myocyte hypertrophy and interstitial fibrosis, are nonspecific and indistinguishable from those in failing muscle in ischemic or valvular heart disease (see Fig. 5-3B). Moreover, the severity of the morphologic changes does not necessarily correlate with the severity of dysfunction or the patient’s prognosis.
Dilated cardiomyopathy has a genetic and often familial basis in approximately 25 to 50% of cases, and our knowledge of the relevant molecular biology is increasing rapidly.10 Mutations most commonly involve genes encoding proteins of the cardiac myocyte cytoskeleton and sarcomere. Alcoholism, pregnancy-associated nutritional deficiency, and myocarditis can yield a dilated phenotype.11
Hypertrophic cardiomyopathy is characterized macroscopically by massive myocardial hypertrophy, usually without dilation (see Fig. 5-3C), and often with disproportionate thickening of the ventricular septum relative to the free wall of the left ventricle (ratio > 1.3) (termed asymmetric septal hypertrophy). In some patients, the basal septum is markedly thickened at the level of the mitral valve, and the outflow of the left ventricle may be narrowed during systole, yielding dynamic left ventricular outflow tract obstruction. In such cases, contact between the left ventricular outflow tract and the anterior mitral leaflet during ventricular systole (observed by echocardiography as systolic anterior motion of the mitral valve) results in outflow tract endocardial thickening, in a configuration that mirrors the anterior leaflet of the mitral valve (see Fig. 5-3D). The most important microscopic features in HCM include (1) disorganized myocytes and contractile elements within cells (myofiber disarray); (2) extreme myocyte hypertrophy, with myocyte diameters frequently more than 40 μm (normal approximately 15 to 20 μm); and (3) interstitial and replacement fibrosis (see Fig. 5-3F).
Hypertrophic cardiomyopathy usually has a genetic basis.12 In many patients the disease is familial; remaining cases are sporadic. Over 1500 mutations have been identified in at least 11 genes; almost all occur in genes for sarcomeric proteins, most commonly β-myosin heavy chain and myosin-binding protein C. Occasional cases mimicking HCM are a result of deposition (eg, Fabry’s disease).
The clinical course of HCM is variable. Complications include atrial fibrillation with potential mural thrombus formation and embolization, infective endocarditis of the mitral valve, intractable cardiac failure, and sudden death. End-stage heart failure can be accompanied by cardiac dilation. Sudden death is common, with risk related to the degree of hypertrophy and specific gene mutations. Reduced stroke volume results from decreased diastolic filling of the massively hypertrophied left ventricle. Patients with left ventricular obstruction may benefit from septal reduction by surgical myotomy/myectomy or chemical ablation.13
Restrictive cardiomyopathy comprises a pathophysiologically heterogeneous spectrum of conditions characterized by heart failure with preserved ejection fraction and previously called diastolic dysfunction; many of the potential causes are functional and are potentiated by aging, obesity, and hypertension.14 Biatrial dilation may be prominent. Structural disorders that interfere with ventricular filling can cause restrictive cardiomyopathy physiology (eg, eosinophilic endomyocardial disease, amyloidosis, storage diseases such as Fabry’s disease, or postirradiation fibrosis, constrictive pericarditis, and HCM). Distinct morphologic patterns may be revealed by endomyocardial biopsy.
Arrhythmogenic right ventricular cardiomyopathy is characterized by a dilated right ventricular chamber and severely thinned right ventricular wall, with extensive fatty infiltration, loss of myocytes with compensatory myocyte hypertrophy, and interstitial fibrosis (see Fig. 5-3G-H).15 Clinical features include right-sided heart failure and arrhythmias. Arrhythmias are often brought on by exertion, and this condition is associated with sudden death in athletes. ARVC may be associated with mutations in several genes involved in cell-cell adhesion and in intracellular signaling.
Myocardial ischemia occurs when perfusion via coronary flow is inadequate to meet metabolic needs, thus interfering with both the cellular delivery of oxygen and nutrients to cardiac myocytes, and the removal of waste products. Myocardial ischemia most often results from obstruction or narrowing of a coronary artery secondary to atherosclerosis. Nonatherosclerotic epicardial coronary artery obstructions can also occur in autoimmune diseases (eg, systemic lupus erythematosus and rheumatoid arthritis), progressive systemic sclerosis (scleroderma), vasculitis (eg, Buerger disease and Kawasaki disease), fibromuscular dysplasia, and as a result of dissection, spasm, embolism, or some drugs such as cocaine. Obstruction of small intramural coronary arteries occurs in diabetes, Fabry’s disease, and amyloidosis, and in cardiac allografts (see later). Decreased coronary flow leading to global hypoperfusion and myocardial ischemia can occur during cardiopulmonary bypass. Ischemia can also result from increased cardiac demand secondary to exercise, tachycardia, hyperthyroidism, or ventricular hypertrophy and/or dilation. Moreover, the effects of ischemia are potentiated when oxygen supply is decreased secondary to anemia, hypoxia, or cardiac failure.
Atherosclerosis is a chronic, progressive, multifocal disease of the vessel wall, beginning in the intima, whose characteristic lesion is the atheroma or plaque that forms through intimal thickening (mediated predominantly by smooth muscle cell proliferation and matrix production) and lipid accumulation (mediated primarily by insudation of lipid into the arterial wall).16 Atherosclerosis primarily affects the large elastic arteries and large- and medium-sized muscular arteries of the systemic circulation, particularly near branches, sharp curvatures, and bifurcations. Coronary arterial atherosclerosis involves especially the epicardial branches of the left anterior descending (LAD) and circumflex arteries, and the right coronary diffusely, but generally not their intramural branches. Most atheromas in the coronary arteries are segmental and eccentric, with plaque-free segments longitudinally and circumferentially. In early lesions, the plaque bulges outward at the expense of the media with the arterial lumen remaining circular in cross-section at essentially the same original diameter (ie, the vessel wall outer diameter enlarges, a process termed vascular remodeling).17
Although veins are usually spared from atherosclerosis, venous grafts interposed within branches of the arterial system (such as aorto-coronary bypass grafts created from saphenous vein) frequently develop intimal thickening and ultimately atherosclerosis, with obstructions or aneurysms. Paradoxically, some arteries used as arterial grafts, including the internal mammary artery (IMA), are largely spared.
The prevailing theory of lesion formation in atherosclerosis centers on interactions among arterial wall endothelial and vascular smooth muscle cells, circulating monocytes, platelets, and plasma lipoproteins. A key contributor to atherosclerosis is endothelial cell injury induced by chronic hypercholesterolemia, homocystinemia, chemicals in cigarette smoke, viruses, localized hemodynamic forces, systemic hypertension, hyperglycemia, or the local effects of cytokines. These factors cause phenotypic and hence functional changes in endothelial cells, called endothelial dysfunction.18 Endothelial dysfunction causes (1) vasoconstriction owing to decreased production of the vasodilator nitric oxide; (2) increased permeability to lipoproteins; (3) expression of tissue factor leading to thrombosis; and (4) expression of certain injury-induced adhesion molecules leading to adherence of platelets and inflammatory cells.
Progression from an early, subendothelial lesion (called a fatty streak) to a complex atheromatous plaque involves the following processes: (1) monocytes adhere to endothelial cells, migrate into the subendothelial space, and transform into tissue macrophages; (2) smooth muscle cells migrate from the media into the intima, proliferate, and secrete collagen and other extracellular matrix (ECM) constituents; (3) lipids accumulate via phagocytosis in macrophages (forming foam cells) and smooth muscle cells, as well as extracellularly; (4) lipoproteins are oxidized in the vessel wall leading to generation of potent biologic stimuli such as chemoattractants and cytotoxins; (5) persistent chronic inflammation; (6) cellular necrosis with release of intracellular lipids (mostly cholesterol esters); and often (7) calcification. Mature atherosclerotic plaques consist of a central core of lipid, cholesterol crystals, macrophages, smooth muscle cells, foam cells, and lymphocytes along with necrotic debris, separated from the lumen by a fibrous cap rich in collagen.
Clinical manifestations of advanced coronary arterial atherosclerosis occur through encroachment of the lumen leading to progressive stenosis, or to acute plaque disruption with thrombosis (see the following). In the absence of significant coronary blockages, myocardial perfusion is adequate at rest, and compensatory vasodilation provides flow reserve that is more than sufficient to accommodate increased metabolic demand during vigorous exertion. When the coronary luminal cross-sectional area is decreased by approximately 75%, blood flow becomes limited during exertion; with 90% reduction, coronary flow may be inadequate at rest. However, occlusions that develop slowly may stimulate the formation of collateral vessels that protect against distal myocardial ischemia. Aneurysms may form secondary to atherosclerosis as a result of destruction of the media beneath a plaque, a process most common in the aorta and other large vessels (where plaque does not easily cause obstruction). The natural history, morphologic features, key pathogenetic events, and clinical complications of atherosclerosis are summarized in Figs. 5-4 and 5-5.
FIGURE 5-4
Acute plaque rupture with superimposed thrombus complicating coronary arterial atherosclerosis and triggering fatal myocardial infarction. (A) Gross photo. (B) Photomicrograph. (The arrow demonstrates the site of plaque rupture.) ([B] Reproduced with permission from Schoen FJ: Interventional and Surgical Cardiovascular Pathology: Clinical Correlations and Basic Principles. Philadelphia: Saunders; 1989.)


FIGURE 5-5
Schematic diagram summarizing the morphology, pathogenesis, and complications of atherosclerosis. Plaques usually develop slowly and insidiously over many years, beginning in childhood or shortly thereafter and exerting clinical effect in middle age or later. As described in the text, lesions may progress from a fatty streak to a fibrous plaque and then to plaque complications that lead to disease. ECM = extracellular matrix; SMC = smooth muscle cell. (Reproduced with permission from Kumar V, Fausto N, Abbas A, et al: Robbins/Cotran Pathologic Basis of Disease, 8th ed. Philadelphia, WB Saunders, 2010.)

The onset and prognosis of ischemic heart disease are not well predicted by the angiographically determined extent and severity of luminal obstructions.19 The conversion of chronic stable angina or an asymptomatic state to an acute coronary syndrome (ie, myocardial infarction, unstable angina, and sudden coronary death) is dependent on dynamic vascular changes, such as fracture or rupture of the fibrous cap, exposing deep plaque constituents, and subsequent thrombus, wither occlusive, or partial, sometimes with emboli. Less commonly, the change is erosion and/or ulceration of the fibrous cap, setting the stage for platelet aggregation and mural or total thrombosis.
Plaques having a high propensity to rupture are known as vulnerable plaques. Such lesions (1) have a thin collagenous fibrous cap and few smooth muscle cells (the cells that produce collagenous matrix); (2) contain many macrophages producing matrix metalloproteinases (MMP) (enzymes that degrade the collagen that ordinarily lends strength to the fibrous cap); and (3) contain large areas of foam cells, extracellular lipid and necrotic debris. Inflammation may contribute to coronary thrombosis by altering the balance between prothrombotic and fibrinolytic properties of the endothelium. There is evidence that lipid lowering by diet or drugs such as statins (HMG CoA reductase inhibitors) reduces accumulation of macrophages expressing matrix-degrading enzymes and thereby stabilizes plaque by increasing the thickness and strength of the fibrous cap.20
Vulnerable plaques without significant obstruction can be clinically important. Indeed, pathologic and clinical studies show that plaques that rupture and lead to coronary occlusion often produced only mild to moderate luminal stenosis (and often no symptoms) prior to acute plaque change. Thus, there is great interest in identifying vulnerable plaques in individuals at a potentially therapeutic stage. Calcification of the coronary arteries detected noninvasively by electron beam computed tomography predicts the extent of atherosclerotic disease overall but does not predict plaque instability. The most relevant features of plaque structure can be evaluated using intravascular ultrasound, optical coherence tomography (OCT), and potentially noninvasive molecular imaging.21
The events that trigger abrupt changes in plaque configuration and superimposed thrombosis and the efficacy and safety of interventional therapies depend on influences both intrinsic (eg, structure and composition, as described above) and extrinsic (eg, blood pressure, vasospasm, and platelet reactivity) to the plaque.22,23 Potential outcomes for ruptured plaques include progression to thrombotic occlusion, nonocclusive thrombosis, healing at the site of plaque erosion, atheroembolization or thromboembolization, organization of mural thrombus causing (plaque progression), and organization of the occlusive mass with recanalization.
The clinical manifestations of ischemic heart disease most frequently reflect the downstream effects of a complex and dynamic interaction among fixed atherosclerotic narrowing of the epicardial coronary arteries, plaque vulnerability, intraluminal thrombosis overlying a ruptured or fissured atherosclerotic plaque, platelet aggregation, vasospasm, and the responses of the myocardium to ischemia.
The changes in the myocardium following the onset of myocardial ischemia are sequential and the cellular consequences are primarily determined by the severity and duration of flow deprivation (Table 5-1). Within seconds of onset, ischemia induces a transition from aerobic metabolism to anaerobic glycolysis in cardiac myocytes, leading to inadequate production of high-energy phosphates such as ATP, and the accumulation of metabolites such as lactic acid, causing intracellular acidosis. Myocardial function is exquisitely sensitive to these biochemical consequences, with total loss of contraction within 2 minutes in severe ischemia. Nevertheless, ischemic changes in an individual cell are not immediately lethal, and short duration injury is potentially reversible. Irreversible injury of cardiac myocytes marked by cell membrane structural defects occurs only after 20 to 40 minutes within the most severely ischemic area (Fig. 5-6). Lethal injury to clusters of cells owing to severe prolonged ischemia causes myocardial infarction.
FIGURE 5-6
Temporal sequence of early biochemical findings and progression of necrosis after onset of severe myocardial ischemia. Left panel: Early changes include loss of ATP and accumulation of lactate. Right panel: Myocardial injury is potentially reversible for approximately 20 minutes after the onset of even the most severe ischemia. Thereafter, progressive loss of viability occurs, which is complete by 6 to 12 hours. The benefits of reperfusion are greatest when it is achieved early; progressively smaller benefit is accrued when reperfusion is delayed.

Event/process | Time of onset |
Onset of anerobic metabolism | Within seconds |
Loss of contractility | <2 min |
ATP* reduced | |
to 50% of normal | 10 min |
to 10% of normal | 40 min |
Irreversible cell injury | 20-40 min |
Microvascular injury | >1 h |
Pathologic feature | Time of recognition |
Ultrastructural features of reversible injury | 5-10 min |
Ultrastructural features of irreversible damage | 20-40 min |
Wavy fibers | 1-3 h |
Staining defect with tetrazolium dye | 2-3 h |
Classic histologic features of necrosis | 6-12 h |
Gross alterations | 12-24 h |
Within the region of myocardium vulnerable to die if ischemia is not relieved in a timely manner (termed the area at risk), loss of perfusion is not uniform and not all cells in the area at risk are equally affected. The most severely affected myocytes, and therefore the first to become necrotic, reside in the subendocardium and in the papillary muscles furthest from lateral regions of noncompromised circulation and adequate perfusion. Thus, if uninterrupted ischemia progresses, there is a wavefront of cell death outward from the mid-subendocardial region, eventually encompassing the lateral borders and less severely ischemic subepicardial and peripheral regions of the area at risk. In myocardial infarction, approximately 50% of the area at risk becomes necrotic in approximately 3 to 4 hours. The final transmural extent of an infarct is generally established within 6 to 12 hours. The key principle is that if perfusion is restored prior to the onset of irreversible changes, cell death can be prevented. Thus, restoration of flow to a severely ischemic area via therapeutic intervention (such as percutaneous coronary intervention [PCI] with stent placement) can alter the outcome, depending on the interval between the onset of ischemia and restoration of blood flow (reperfusion).24
Necrosis existing less than 6 or more hours before patient death is not visible by routine gross or microscopic pathologic analysis of a cardiac specimen at autopsy. However, in a patient who died at least 2 to 3 hours following the onset of gross infarction, the presence of a necrotic region may be detected as a staining defect with triphenyl tetrazolium chloride (TTC), a dye that turns viable myocardium a brick-red color on reaction of the dye with intact myocardial dehydrogenases.25 The earliest observable microscopic features of infarction are intense eosinophilia, nuclear pyknosis, and loss of myocytes in clusters; some may be stretched and wavy. Short-term ischemia without necrosis cannot be reliably demonstrated by pathologic examination.
The inflammation and repair sequence in response to myocardial cell death follows a largely stereotyped sequence similar to tissue repair following injury at extracardiac sites. The inflammatory reaction is characterized by early exudation of polymorphonuclear leukocytes, seen after 6 to 12 hours and maximal within 1 to 3 days. Subsequently (3 to 5 days), the infiltrate consists predominantly of macrophages that remove the necrotic tissue. Collagen production accompanied by neovascularization begins approximately 7 to 10 days at the margins of preserved tissue. The gross appearance reflects the progressive microscopic changes described above (Fig. 5-7). Ultimately, the infarcted tissue is replaced by dense collagenous scar, which is fully mature by about 6 to 8 weeks. Healing of myocardial infarction may be altered by reperfusion (see the following), mechanical stress, sex, and neurohumoral and other factors such as immunosuppressive drugs.26
Although cardiac myocytes are traditionally thought incapable of regeneration, a growing body of evidence suggests that regeneration of cardiac myocytes can occur under certain circumstances, including at the viable borders of myocardial infarcts.27 Whether this capacity for renewal can be harnessed to therapeutic advantage is not yet known (discussed later in this chapter).
Reperfusion of an ischemic zone occurring before the onset of irreversible injury (recall, approximately 20 to 30 minutes) prevents infarction. Reperfusion later (ie, following some cell death) may limit infarct size through salvage of myocytes located outside the leading edge of the “wavefront,” provided that these myocytes are only reversibly injured at the time reperfusion occurs.28 Thus, the potential for recovery of viable tissue decreases with increasing severity and duration of ischemia (see Fig. 5-6). Owing to the typical progression of ischemic injury, reperfusion 3 to 4 hours following onset of ischemia is considered the practical limit for significant myocardial salvage, and 90 minutes (“door to balloon time”) is the present clinical goal in PCI.29
Reperfused previously ischemic myocardium often has hemorrhage (owing to microvascular damage; see the following) and necrotic myocytes with transverse eosinophilic lines (called contraction bands) that represent hypercontracted sarcomeres (Fig. 5-8) caused by ischemic cell membrane damage followed by a massive cellular influx of calcium derived from the restored blood flow. Microvascular occlusions (resulting from endothelial or interstitial edema and/or plugging by platelet or neutrophil aggregates) may inhibit the reperfusion of damaged regions (no-reflow phenomenon).30 Moreover, reperfusion itself may damage some of the ischemic but still viable myocytes that were not irreversibly injured (called reperfusion injury), and arrhythmias may occur.31 In reperfusion after severe global ischemia (eg, during cardiac surgery), the left ventricle may undergo a massive tetanic contraction (stone heart syndrome).32
FIGURE 5-8
Reperfusion following severe myocardial ischemia. (A) Large, densely hemorrhagic anteroseptal acute myocardial infarct from patient treated by intracoronary thrombolysis for left anterior descending (LAD) artery thrombus, approximately 4 hours following onset. (B) Subendocardial circumferential hemorrhagic acute myocardial necrosis occurring perioperatively in cardiac valve replacement. (C) Photomicrograph of hemorrhagic myocardial necrosis. (D) High-power photomicrograph demonstrating contraction bands (arrow). Hematoxylin and eosin 375×.




Although reperfusion may salvage ischemic but not necrotic myocardium, metabolic and functional recovery is usually not instantaneous; indeed, reversible postischemic myocardial dysfunction (called myocardial stunning) may persist for hours to days following brief periods of ischemia.33 Myocardial stunning may also occur in the setting of PCI, cardiopulmonary bypass, or ischemia related to unstable angina or stress.
Regions of viable myocardium with chronically reduced coronary blood flow may have impaired function (termed hibernating myocardium).34,35 Myocardial hibernation is characterized by (1) persistent wall motion abnormality, (2) low myocardial blood flow, and (3) evidence of viability in at least some of the affected areas. Contractile function of hibernating myocardium can improve if blood flow returns toward normal or if oxygen demand is reduced. Correction of this abnormality is likely responsible for the reversal of long-standing defects in ventricular wall motion observed following coronary bypass graft surgery or PCI. Morphologically, sublethal chronic ischemic injury often manifests as myocyte vacuolization, particularly in the subendocardium.36
Adaptation to short-term transient ischemia (ie, duration insufficient to cause cell death) may induce tolerance against subsequent, more severe ischemic insults (ischemic preconditioning).37 Thus, short (eg, 5-minute) periods of cardiac ischemia followed by reperfusion can protect myocardium against injury during a prolonged period of subsequent ischemia. Understanding the yet uncertain mechanisms of this protection could lead to targets for “preemptive” pharmacologic stimulation of these pathways.
Coronary atherosclerosis with acute plaque rupture and superimposed thrombosis often results in a transmural (Q-wave) myocardial infarct, in which ischemic necrosis involves at least half and usually a nearly full thickness of the ventricular wall in the distribution of the involved artery. In contrast, a subendocardial (nontransmural, non–Q-wave) infarct constitutes an area of ischemic necrosis that is limited to the inner third to half of the ventricular wall, which can occur in the setting of episodic hypotension, global ischemia, or hypoxemia, or from interruption by reperfusion of the evolution of a transmural infarct. Subendocardial infarction associated with diffuse stenosing coronary atherosclerosis can be multifocal, extending laterally beyond the perfusion territory of a single coronary artery.
The short-term in-hospital mortality rate from acute myocardial infarction has declined from nearly 30% in the 1950s and 1960s to 7% or less today, especially for patients who receive aggressive reperfusion/revascularization and pharmacologic therapy.38 However, half of all deaths from myocardial infarction occur within the first hour after the onset of symptoms, potentially before a victim can reach the hospital. Poor prognostic factors include advanced age, female sex, diabetes mellitus, and a previous myocardial infarction. Left ventricular function and the extent of obstructive lesions in vessels perfusing viable myocardium are the most important prognostic factors.
Important complications of myocardial infarction include ventricular dysfunction, cardiogenic shock, arrhythmias, cardiac rupture, infarct extension and expansion, papillary muscle dysfunction, right ventricular involvement, ventricular aneurysm, pericarditis, and systemic arterial embolism; the cardiac rupture syndromes and ventricular aneurysm are illustrated in Fig. 5-9. Outcome after myocardial infarction depends on infarct size, location, and transmurality. Patients with transmural anterior infarcts are at greatest risk for regional dilation and mural thrombi and have a worse clinical course than those with inferior-posterior infarcts. In contrast, inferior-posterior infarcts are more likely to have serious conduction blocks and right ventricular involvement.
FIGURE 5-9
Cardiac rupture syndromes and ventricular aneurysm following myocardial infarction. (A) Anterior myocardial rupture (arrow). (B) Rupture of the ventricular septum (arrow). (C) Rupture of a necrotic papillary muscle (arrow). (D) Large left ventricular aneurysm, with thin fibrotic wall (arrow) and mural thrombus.




Myocardial infarcts produce functional abnormalities approximately proportional to their size. Large infarcts have a higher probability of cardiogenic shock and congestive heart failure. Nonfunctional scar tissue resulting from previous infarcts and areas of stunned or hibernating myocardium may also contribute to overall ventricular dysfunction. Cardiogenic shock following myocardial infarction generally indicates a large infarct (often > 40% of the left ventricle). The high mortality of myocardial dysfunction and cardiogenic shock has been alleviated somewhat by the use of ventricular assist devices (VADs) to bridge patients through phases of prolonged reversible ischemic dysfunction.
Although many patients have cardiac rhythm abnormalities following myocardial infarction, the conduction system is involved by necrosis or inflammation only in a minority, and heart block following myocardial infarction usually is transient. Tachyarrhythmias usually originate owing to electrically unstable ischemic or necrotic myocardium, often at the edge of an infarct. However, autopsy studies of sudden death victims and clinical studies of resuscitated survivors of cardiac arrest show that only a minority of patients with ischemia-induced malignant ventricular arrhythmias develop a full-blown acute myocardial infarction.
Cardiac rupture syndromes comprise three entities (see Fig. 5-9A-C): (1) rupture of the ventricular free wall (most common), usually with hemopericardium and cardiac tamponade; (2) rupture of the ventricular septum (less common), leading to an acquired ventricular septal defect with a left-to-right shunt; and (3) papillary muscle rupture (least common), resulting in the acute onset of severe mitral regurgitation. Ruptures tend to occur relatively early following infarction with as many as 25% presenting within 24 hours (mean interval 4 to 5 days), most commonly through the lateral free wall. Acute free wall ruptures usually are rapidly fatal; repair is rarely possible.39 Rarely, a rupture is contained as a hematoma communicating with the ventricular cavity (false aneurysm); this is an unstable situation and the false aneurysm may eventually rupture.
Postinfarction septal ruptures with acute ventricular septal defect are of two types: (1) single or multiple sharply localized, jagged, linear passageways that connect the ventricular chambers (simple type), usually involving the anteroapical aspect of the septum; and (2) defects that tunnel serpiginously through the septum to a somewhat distant opening on the right side (complex type), usually involving the basal inferoseptal wall.40 In complex lesions, the tract may extend into regions remote from the site of the infarct, such as the right ventricular free wall. Without surgery, the prognosis is poor for patients with infarct-related ventricular septal defects.
Papillary muscles are particularly vulnerable to ischemic injury and rupture, particularly the posterior medial papillary muscle, and rupture can occur later than other rupture syndromes (as late as 1 month after myocardial infarction). Because tendinous cords arise from the heads of the papillary muscles and cords from each are distributed to both valve leaflets, interference with the structure or function of either papillary muscle can result in dysfunction of both mitral valve leaflets and resultant mitral regurgitation (see later).
Isolated right ventricular infarction and involvement of the right ventricle by extension of a posteroseptal infarct can have important functional consequences, including right ventricular failure with or without tricuspid regurgitation and arrhythmias.
Infarct extension is characterized by incremental new or recurrent necrosis in the same distribution as a completed recent infarct. Extension most often occurs along the lateral and subepicardial borders of a recent infarct, and histologically appears younger than the previously necrotic myocardium. In contrast, infarct expansion is a disproportionate thinning and dilation of the infarcted region which does not lead to additional necrotic myocardium per se, but may promote both further ischemia and intracardiac mural thrombus formation. The increase in ventricular volume caused by regional dilation increases the wall stress and thereby the workload of noninfarcted myocardium. Infarct expansion is often the substrate for late aneurysm formation and increases morbidity and mortality.
Ventricular aneurysms are large scars that paradoxically bulge during ventricular systole and often result from healing of a large transmural infarct that undergoes expansion (see Fig. 5-9D).41 Although frequently as thin as 1 mm, ventricular aneurysms rarely rupture owing to their walls of tough fibrous or fibrocalcific tissue. Hypertrophied myocardial remnants as well as necrotic but inadequately healed myocardium often are present in aneurysm walls and mural thrombus is common.
Ventricular remodeling comprises the collective structural changes that occur in both the necrotic zone and uninvolved areas of the heart, including left ventricular dilation, wall thinning by infarct expansion, compensatory hypertrophy of noninfarcted myocardium, and potentially late aneurysm formation.42 Congestive heart failure secondary to coronary artery disease (CAD) occurs when the overall function of viable myocardium can no longer maintain an adequate cardiac output or regions of hyperfunctioning residual myocardium suffer additional ischemic episodes.
Revascularization early after acute myocardial infarction is rationalized as follows: (1) prolonged thrombotic occlusion of a coronary artery causes transmural infarction; (2) the extent of necrosis during an evolving myocardial infarction progresses as a wavefront and becomes complete only 6 to 12 hours or more following coronary occlusion (see Fig. 5-6); (3) both early- and long-term mortality following acute myocardial infarction correlate strongly with the amount of residual functioning myocardium; and (4) early reperfusion rescues some jeopardized myocardium.43 Thus, the benefits of revascularization depend on and are assessed by the amount of myocardium salvaged, recovery of left ventricular function, and resultant reduction in mortality. These clinical end points are largely determined by the time interval between onset of symptoms and successful reflow, and the degree of residual stenosis of the infarct vessel. Spontaneous recanalization via endogenous thrombolysis can be beneficial to left ventricular function but occurs in only a small percentage of patients within the critical interval.
Percutaneous coronary intervention can restore blood flow through a diseased portion of the coronary circulation obstructed by atherosclerotic plaque and/or thrombotic deposits, and in obstructions in saphenous vein grafts, IMA grafts, and occasionally, coronary arteries in transplanted hearts.
In percutaneous transluminal coronary angioplasty (PTCA), the plaque splits at its weakest point and enlargement of the lumen occurs by plaque fracture (the predominant mechanism); and by embolization, compression, redistribution of the plaque contents, and overall mechanical expansion of the vessel wall can also occur.44 The split extends to the intimal-medial border and often into the media, is accompanied by variable circumferential and longitudinal medial dissection, and can induce a flap that impinges on the lumen. These changes can result in local flow abnormalities and generation of new, thrombogenic blood-contacting surfaces (to some extent similar to what is observed with spontaneously disrupted plaque) and contribute to the propensity for acute thrombotic closure.
The long-term success of PTCA is limited by the development of progressive restenosis, which occurs in 30 to 50% of patients, most frequently within the first 4 to 6 months.45 Although vessel wall recoil and organization of thrombus likely contribute, the major process leading to restenosis is excessive medial smooth muscle migration to the intima, proliferation, and secretion of abundant ECM as a response to angioplasty-induced injury.
Although not widely used today, coronary atherectomy of primary or restenosis lesions mechanically can remove obstructive tissue by excision.46 Deep arterial resection, including medial and even adventitial elements, occurs frequently but has not been associated with acute symptomatic complications. The morphology of arterial vessel healing after directional or rotational atherectomy is similar to that following angioplasty.
Stents are expandable tubes of metallic or polymeric mesh that are used to split open the vessel wall at the site of balloon angioplasty and thereby mitigate the negative sequelae of PTCA.47 Stents preserve luminal patency and provide a larger and more regular lumen by acting as a scaffold to support the disrupted vascular wall and minimize flow disruption and thrombus formation. Placement of a stent yields outcomes superior to angioplasty alone in vessels greater than 3 mm in diameter, chronic total occlusions, stenotic vein grafts, restenotic lesions after angioplasty alone, and in patients with myocardial infarction.48
Stent technology has undergone a rapid evolution, including sequentially: (1) bare-metal stents (BMS); and (2) drug-eluting stents (DES), both used extensively in clinical interventional cardiology; and more recently (3) completely resorbable/biodegradable stents (RBS). Bare-metal stents for coronary implantation are short tubular segments of metal mesh composed of balloon-expandable 316L stainless steel or nickel-titanium alloy (Nitinol) that range from approximately 2.5 to 3.5 mm in diameter and approximately 1 to 3 cm in length. Development has focused on permitting stents to become more flexible and more easily delivered and deployed, allowing the treatment of a greater number and variety of lesions.
Key stent complications are thrombosis, usually occurring early, and late proliferative restenosis (Fig. 5-10). Thrombotic occlusion, occurring in 1 to 3% of patients within 7 to 10 days of the procedure (see Fig. 5-10A), has largely been overcome by aggressive multidrug treatment with antiplatelet agents such as clopidogrel, aspirin, and glycoprotein IIb/IIIa inhibitors. The major long-term complication of bare-metal stenting is in-stent restenosis, which occurs in 50% of patients within 6 months.49 The causes of stent thrombosis and restenosis are complex and are largely owing to stent-tissue interactions, damage to the endothelial lining, and stretching of the vessel wall, which stimulate inflammation and adherence and accumulation of platelets and fibrin.50 Stent wires may eventually become completely embedded in an endothelium-lined intimal fibrosis layer composed of smooth muscle cells in a collagen matrix (see Fig. 5-10B). This tissue may thicken secondary to the release of growth factors, chemotactic factors, and inflammatory mediators from platelets and other inflammatory cells that result in proliferation of smooth muscle cells and increased production of ECM molecules, narrowing the lumen and resulting in restenosis.
FIGURE 5-10
Stent pathology. (A) Thrombosis. H&E stain. (B) Thickened proliferative neointima separating the stent wires (black structure) from the lumen, with bare metal coronary artery stent implanted long term. Movat stain. (Reproduced with permission from Silver MD, Gotlieb AI, Schoen FJ: Cardiovascular Pathology, 3rd ed. Philadelphia: Churchill Livingstone/Elsevier; 2001.)


Drug-eluting stents, which impart the controlled release of drugs from durable polymers to the vessel wall, effectively inhibit in-stent restenosis.51,52 The drugs used most widely are rapamycin (sirolimus)53 and paclitaxel54 in the Cypher (Cordis) and Taxus (Boston Scientific) stents, respectively. Rapamycin, a drug used for immunosuppression in solid organ transplant recipients, inhibits proliferation, migration, and growth of smooth muscle cells and ECM synthesis. Paclitaxel, a drug used in the chemotherapeutic regimens for several types of cancer, also has similar anti-smooth muscle cell activities. These drugs are embedded in a polymer matrix (such as a copolymer of poly-n-butyl methacrylate and polyethylene-vinyl acetate or a gelatin-chondroitin sulfate coacervate film) that is coated onto the stent. However, stent thrombosis emerged as a major safety concern with DES early after their adoption in clinical practice, requiring prolonged dual antiplatelet therapy.55 Pathological examination of clinical specimens and animal studies have suggested that these DES were associated with DES-induced inhibition of stent endothelialization and delayed arterial healing and polymer hypersensitivity reactions resulting in chronic inflammation that contributed to stent thrombosis. Recently, DES have been developed to overcome these issues with improved stent designs and construction and the use of biocompatible polymers.56
In contrast to the permanent presence of a foreign body in BMS and DES, RBS provide a scaffold and then ultimately disappear by resorption of foreign scaffold material that may potentiate a thrombotic event, permit more versatility in subsequent therapies, and not interfere with the diagnostic evaluation by noninvasive imaging such as cardiac magnetic resonance and CT.57,58 Several RBS are in development or in clinical trials. The key challenge is to balance biomechanics (strength, deliverability, and lesion crossing), potential for side-branch occlusion (owing to thicker struts), durability and biocompatibility, and to control the kinetics of stent degradation at a rate appropriate to maintain mechanical strength to limit recoil.
Coronary artery bypass graft (CABG) surgery improves survival in patients with significant left main CAD, three-vessel (and possibly two-vessel) disease, or reduced ventricular function, and prolongs and improves the quality of life in patients with left main equivalent disease (proximal LAD and proximal left circumflex), but does not protect them from the risk of subsequent myocardial infarction.59 The principal mechanism for these benefits is thought to be the restoration of blood flow to hibernating myocardium.
The hospital mortality rate for CABG surgery is approximately 1% in low-risk patients, with fewer than 3% of patients suffering perioperative myocardial infarction. The most consistent predictors of mortality after CABG are urgency of operation, age, prior cardiac surgery, female sex, low left ventricular ejection fraction, degree of left main stenosis, and number of vessels with significant stenoses. The most common mode of early death after CABG is acute cardiac failure leading to low output or arrhythmias, owing to myocardial necrosis (often with features of reperfusion described in the preceding), postischemic dysfunction of viable myocardium, or a metabolic cause, such as hypokalemia.
Early thrombotic occlusion of the graft vessel may occur, usually potentiated by inadequate distal run-off from extremely small and/or atherosclerotic distal native coronaries. Additional factors may include atherosclerosis, arterial branching or dissection of blood into the graft or native vessel at the anastomotic site, or distortion of a graft that is too short or too long for the intended bypass. In some cases, thrombosis occurring early postoperatively involves only the distal portion of the graft, suggesting that early graft thrombosis was initiated at the distal anastomosis. Most patients who die early after CABG have patent grafts.
The patency of saphenous vein grafts is reported as 60% at 10 years; occlusion results from (with increasing postoperative interval) thrombosis, progressive intimal thickening, and/or obstructive atherosclerosis.60 Between 1 month and approximately 1 year, graft stenosis is usually caused by intimal hyperplasia with excessive smooth muscle proliferation and ECM production (similar to that seen in restenosis following angioplasty and stenting). Atherosclerosis becomes the predominant mechanism in graft occlusion beyond 1 to 3 years after CABG, and earliest in those patients with the most significant atherosclerotic risk factors. Plaques in grafts often have poorly developed fibrous caps with large necrotic cores and can develop secondary dystrophic calcific deposits that extend to the lumen (Fig. 5-11); thus, the potential for disruption, aneurysmal dilation, and embolization of atherosclerotic lesions in vein grafts exceeds that for native coronary atherosclerotic lesions, and balloon angioplasty, stenting or intraoperative manipulation of grafts may potentiate atheroembolism.
FIGURE 5-11
Atherosclerosis of saphenous vein bypass graft. (A) Fibrous cap (between arrows) is attenuated over the necrotic core (large asterisk). Lumen is at upper right. (B) Saphenous vein graft aneurysm. Hematoxylin and eosin (A) 100×; Gross photo. Verhoff von Giesen stain (for elastin) 10×. ([A] Reproduced with permission from Schoen FJ: Interventional and Surgical Cardiovascular Pathology: Clinical Correlations and Basic Principles. Philadelphia: Saunders; 1989. [B] Reproduced with permission from Liang BT, Antman EM, Taus R, et al: Atherosclerotic aneurysms of aortocoronary vein grafts, Am J Cardiol. 1988 Jan 1;61(1):185-188.)


In contrast to saphenous vein grafts, IMA grafts have greater than 90% patency at 10 years (Fig. 5-12).61 Multiple factors likely contribute to the remarkably higher long-term patency of IMA grafts compared with vein grafts. Although free saphenous vein grafts sustain disruption of their vasa vasora and nerves, endothelial damage, medial ischemia, and acutely increased internal pressure, an IMA graft generally has minimal preexisting atherosclerosis and requires minimal surgical manipulation, maintains its nutrient blood supply, is adapted to arterial pressures, needs no proximal anastomosis, and has an artery-to-artery distal anastomosis. Graft and recipient vessel have comparable sizes with the IMA but are disparate (graft substantially larger) with saphenous vein. The advent of off-pump and minimally invasive coronary artery bypass grafting has stimulated efforts to facilitate sutureless anastomosis of the graft to the aorta.62
FIGURE 5-12
Internal mammary artery as coronary artery bypass graft removed 13 years following surgery, demonstrating near-normal morphology, including an intact internal elastic lamina (arrow) Verhoff von Giesen stain (for elastin, black) 60×. (Reproduced with permission from Schoen FJ: Interventional and Surgical Cardiovascular Pathology: Clinical Correlations and Basic Principles. Philadelphia: Saunders; 1989.)

Although advances in medical therapy and PCI have contributed to fewer CABGs performed each year, challenges remain and new technologies are under consideration to make the procedure safer and more efficacious.63 For example, patients needing revascularization have a much more complicated combination of disease processes and many of the patients have extensive CAD with prior attempts at revascularization. The future of coronary artery bypass grafting may benefit from smaller incisions and potentially robotic endoscopic CABG, facilitated by novel anastomotic devices and intraoperative determination of graft patency. Hybrid surgical/catheterization suites that allow for simultaneous staged CABG and PCI are currently under development.
Normal valve function requires structural integrity and coordinated interactions among multiple anatomical components. For the atrioventricular valves (mitral and tricuspid), these elements include leaflets, commissures, annulus, tendinous cords (chordae tendineae), papillary muscles, and the atrial and ventricular myocardium. For the semilunar valves (aortic and pulmonary), the key structures are the cusps, commissures, and their respective supporting structures in the aortic and pulmonary roots.
The anatomy of the mitral and aortic valves is illustrated in Fig. 5-13.
FIGURE 5-13
Normal mitral and aortic valves. In (A), opened left ventricle of the normal heart, demonstrating mitral valve and components of the mitral apparatus. (B) Aortic valve viewed from distal aspect in open (bottom) and closed (top) phases. (C) Normal aortic valve histology, demonstrating layered structure, including the fibrosa (f), spongiosa (s), and ventricularis (v) layers. The inflow surface is at bottom. Verhoeff van Giesen (stain for elastin, black) 150×. ([A] Reproduced with permission from Schoen FJ: Interventional and Surgical Cardiovascular Pathology: Clinical Correlations and Basic Principles. Philadelphia: Saunders; 1989. [B and C] Reproduced with permission from Silver MD, Gotlieb AI, Schoen FJ: Cardiovascular Pathology, 3rd ed. New York: Churchill Livingstone/Elsevier; 2001.)




The mitral valve (see Fig. 5-13A) has two leaflets: the anterior (also called septal, or aortic) leaflet, roughly triangular and deep, with the base inserting on approximately one-third of the annulus, and the posterior (also called mural or ventricular) leaflet, more shallow than the anterior and attached to about two-thirds of the annulus. The posterior leaflet typically has distinct scallops that are designated P1, P2, and P3, respectively, beginning from the anterolateral toward the posteromedial commissure. The mitral leaflets have a combined area approximately twice that of the annulus; apposition during systole occurs over approximately 50% of the depth of the posterior leaflet and 30% that of the anterior leaflet. Each leaflet receives tendinous cords from both anterior and posterior papillary muscles. The mitral valve orifice is D-shaped, with the flat anteromedial portion comprising the subaortic attachment of the anterior mitral leaflet. This part of the annulus is fibrous and noncontractile; in contrast, the posterolateral portion of the annulus is muscular and contracts during systole to asymmetrically reduce the area of the orifice. The edges of the mitral leaflets are held in or below the plane of the orifice by the tendinous cords, which themselves are pulled from below by the contracting papillary muscles during systole. This serves to draw the leaflets to closure and maintain competence. The posterior leaflet, with its more delicate structure and shorter annulus-to-free-edge dimension than the anterior, is more prone to postinflammatory fibrous retraction and deformation owing to myxomatous degeneration. The orifice of the tricuspid valve is larger and less distinct than that of the mitral; its three leaflets (anterior, posterior, and septal) are larger and thinner than those of the mitral valve.
The aortic valve has structural complexity at several levels.64 The three aortic valve cusps (left, right, and noncoronary) attach to the aortic wall in a semilunar fashion, ascending to the commissures and descending to the base of each cusp (see Fig. 5-13B). Commissures are spaced approximately 120 degrees apart and occupy the three points of the annular crown, representing the sites of separation between adjacent cusps. Behind the valve cusps are dilated pockets of aortic root, called the sinuses of Valsalva. The right and left coronary arteries arise from orifices behind the right and left cusps, respectively. At the midpoint of the free edge of each cusp is a fibrous nodule called the nodule of Arantius. A thin, crescent-shaped portion of the cusp on either side of the nodule, termed the lunula, defines the surfaces of apposition of the cusps when the valve is closed (approximately 40% of the separating area). The lunular tissue does not contribute to separate aortic from ventricular blood during diastole. Thus, fenestrations (holes) near the free edges commonly occur as a small (<2 mm in diameter), developmental, or degenerative abnormality and have no functional significance. In contrast, defects in the portion of the cusp below the lunula are associated with functional incompetence; such holes also suggest previous or active infection. When the aortic valve is closed during diastole, there is a back pressure on the cusps of approximately 80 mm Hg. The pulmonary valve cusps and surrounding tissues have architectural similarity to but are more delicate than those of the corresponding aortic components, and lack coronary arterial origins.
All four cardiac valves have a similar microscopically inhomogeneous architecture, consisting of well-defined tissue layers in the plane of the cusp. Using the aortic valve as the paradigm (see Fig. 5-13C), beneath the valvular endothelium, on the inflow side the ventricularis faces the left ventricular chamber and is enriched in radially aligned elastic fibers, which enable the cusps to have minimal surface area when the valve is open but stretch during diastole to form a large coaptation area. The spongiosa is centrally located and is composed of loosely arranged collagen and abundant proteoglycans. This layer has negligible structural strength, but accommodates relative movement between layers during the cardiac cycle and absorbs shock during closure. The fibrosa provides structural integrity and mechanical stability through a dense aggregate of circumferentially aligned, densely packed collagen fibers, largely arranged parallel to the cuspal free edge. Normal human aortic and pulmonary valve cusps have few blood vessels; they are sufficiently thin to be perfused from the surrounding blood. In contrast, the mitral and tricuspid leaflets contain a few capillaries in their most basal thirds.
Best developed in aortic valve, key specializations facilitating valve function include crimp of collagen fibers along their length, bundles of collagen in the fibrous layer oriented toward the commissures, and grossly visible corrugations; these allow cusps to be extremely soft and pliable when unloaded in systole, but taut and stiff when stretched in diastole. Moreover, the orientation of connective tissue and other architectural elements is nonrandom in the plane of the cusp, yielding greater compliance in the radial than circumferential direction. The fibrous network within the cusps effectively transfers the stresses of the closed phase to the annulus and aortic wall. This minimizes sagging of the cusp centers, preserves maximum coaptation, and prevents regurgitation. Additionally, for the mitral valve, the subvalvular apparatus including tendinous cords and papillary muscles is a critical mechanism of valve competency.
Recent studies have fostered an emerging picture of how valves form embryologically, mature in the fetus, and function, adapt, maintain homeostasis, and change throughout life. These essential relationships facilitate an understanding of valve pathology and mechanisms of disease, foster the development of improved tissue heart valve substitutes, and inform innovative approaches to heart valve repair and regeneration.65
During normal development of the heart, the heart tube undergoes looping, following which the valve cusps/leaflets originate from mesenchymal outgrowths known as endocardial cushions.66 A subset of endothelial cells in the cushion-forming area, driven by a complex array of signals from the underlying myocardium, changes their phenotype to mesenchymal cells and migrates into the acellular ECM called cardiac jelly. Likely regulated by TGF-β and vascular endothelial growth factor (VEGF), the transformation of endocardial cells to mesenchymal cells is termed transdifferentiation or endothelial-to-mesenchymal transformation (EMT). Changes in cell phenotype and ECM remodeling continue throughout human fetal and postnatal development, and throughout life, leading to ongoing changes in properties, as evidenced by increasing valve stiffness with increasing age.67,68
Two types of cells are present in the aortic valve: endothelial cells located superficially and interstitial cells located deep to the surface. Aortic valve endothelial cells (VECs) have a different phenotype than endothelial cells in the adjacent aorta and elsewhere,69,70 but the implications of these differences are not yet known. The second cell type comprises the valvular interstitial cells (VICs), which have variable properties of fibroblasts, smooth muscle cells, and myofibroblasts. VICs maintain the valvular ECM, the key determinant of valve durability. To maintain integrity and pliability throughout life, the valve cusps and leaflets must undergo ongoing physiologic remodeling that entails synthesis, degradation, and reorganization of its ECM, which depends on matrix-degrading enzymes such as MMP. Although VICs are predominantly fibroblast-like in normal valves, they can become activated when exposed to environmental (ie, mechanical and chemical) stimulation and assume a myofibroblast-like phenotype that mediates connective tissue remodeling.
Cardiac valve operations utilizing replacement or repair usually are undertaken for dysfunction caused by calcification, fibrosis, fusion, retraction, perforation, rupture, stretching, infection, dilation, or congenital malformations of the valve leaflets/cusps or associated structures. Valvular stenosis, defined as inhibition of forward flow secondary to obstruction caused by failure of a valve to open completely, is almost always caused by a cuspal abnormality that induces a chronic disease process. In contrast, valvular insufficiency, defined as reverse flow caused by failure of a valve to close completely, may result from intrinsic disease of the cusps/leaflets and/or damage to or distortion of the supporting structures (eg, the aorta, mitral annulus, chordae tendineae, papillary muscles, and ventricular free wall). Thus, regurgitation can appear either precipitously, as with cordal rupture, or gradually, as with leaflet scarring and retraction. Both stenosis and insufficiency can coexist in a single valve. The most commonly encountered types of valvular heart disease are illustrated in Figs. 5-14 and 5-15.
FIGURE 5-14
Calcific aortic valve stenosis. (A) Calcific aortic valve disease causing aortic stenosis in an elderly patient, characterized by mineral deposits at basal aspect of cusps. (B) Calcification of congenitally bicuspid aortic valve, having two unequal cusps, the larger with a central raphe (arrow). (C and D) Photomicrographs of calcific deposits in calcific aortic valve disease. Hematoxylin and eosin 15×. Calcific aortic valve stenosis. (C) Nearly transmural deposits with only thin uninvolved cusp on inflow surface (at bottom). (D) Bone formation (osseous metaplasia).




FIGURE 5-15
Etiologies of mitral valvular disease. (A and B) Rheumatic valve disease. (A) Atrial view and (B) subvalvular aspect of valve from patient with rheumatic mitral stenosis. The valvular changes are severe, including diffuse leaflet fibrosis and commissural fusion and ulceration of the free edges of the valve, as well as prominent subvalvular involvement with distortion (arrow in [B]). (C and D) Myxomatous degeneration of the mitral valve. In (C) there is prolapse into the left atrium of a redundant posterior leaflet (arrow). (D) Surgically resected, markedly redundant myxomatous valve. ([A and B] Reproduced with permission from Schoen FJ: Interventional and Surgical Cardiovascular Pathology: Clinical Correlations and Basic Principles. Philadelphia: Saunders; 1989.)


Aortic stenosis (AS) is the most common valvular heart disease in Western countries and has serious consequences.71 The prevalence of AS increases with age, reaching about 3% after the age of 75 in the United States. Thus, the global burden of AS is expected to double within the next 50 years as life expectancy lengthens. The limited available understanding of AS mechanisms and pathobiology has precluded development of effective noninvasive medical treatments.72 Symptomatic severe AS not treated promptly by corrective surgery has a high mortality as well as high and accelerating symptom burden.
Calcific AS, the most frequent valvular abnormality requiring surgery, is usually the consequence of calcium phosphate deposition in either an anatomically normal aortic valve or in a congenitally bicuspid valve (see Fig. 5-14A,B).73 Stenotic, previously normal tricuspid valves present primarily with calcific aortic valve disease in the seventh to ninth decades of life, while congenitally bicuspid valves with superimposed calcification generally become symptomatic earlier (usually sixth to seventh decades).74
Calcific AS is characterized by heaped-up, calcified masses initiated in the cuspal fibrosa at the points of maximal cusp flexion (the margins of attachment); they protrude distally from the aortic aspect into the sinuses of Valsalva, inhibiting cuspal opening. However, the ventricular surfaces of the cusps usually remain smooth (see Fig. 5-14C). The calcification process generally does not involve the free cuspal edges, appreciable commissural fusion is absent, and the mitral valve generally is uninvolved. Calcified material resembling bone is often present (see Fig. 5-14D). Aortic valve sclerosis comprises a common, earlier, and hemodynamically less significant stage of the calcification process. Nevertheless, aortic sclerosis is associated with an approximately 50% increase in the risk of death from cardiovascular causes, even in the absence of hemodynamically significant obstruction of left ventricular outflow.75
Aortic stenosis induces a pressure gradient across the valve, which may reach 75 to 100 mg Hg in severe cases, necessitating a left ventricular pressure of 200 mg Hg or more to expel blood. Consequently, cardiac output is maintained by the development of pressure-overload left ventricular hypertrophy. The onset of symptoms such as angina, syncope, or heart failure in AS heralds the exhaustion of compensatory cardiac hyperfunction and carries a poor prognosis if not treated by aortic valve replacement (AVR).76 Other complications of calcific AS include embolization that may occur spontaneously or during interventional procedures, hemolysis, infective endocarditis, and extension of the calcific deposits into the ventricular septum causing conduction abnormalities.
Aortic valve calcification has been traditionally considered a wholly degenerative, dystrophic, and passive process. However, recent studies suggest active regulation of calcification in aortic valves, with mechanisms that include inflammation, lipid infiltration, and phenotypic modulation of VIC to an osteoblastic phenotype,77 and risk factors overlapping with those of arterial atherosclerosis. Similarities to atherosclerosis have stimulated interest in the possibility that statin drugs may decrease the rate of AS progression; however, benefit of statins for AS has not been supported by clinical studies.78
Bicuspid aortic valve (BAV) typically has two cusps of unequal size, with the larger (conjoined) cusp having a midline raphe, representing an incomplete separation or congenital fusion of two cusps. Less frequently, the cusps are of equal size (see Fig. 5-14B). Neither stenotic nor symptomatic at birth or throughout early life, BAV are predisposed to accelerated calcification; ultimately, almost all become stenotic. Aortic pathology, including dilation and/or dissection, commonly accompanies BAV. Despite a prevalence of approximately 1%,79 BAV and other congenital valve abnormalities underlie over two-thirds of AS in children and almost 50% in adults. Infrequently, BAV become purely incompetent, or complicated by infective endocarditis, even when the valve is hemodynamically normal. Only rarely is an uncomplicated BAV encountered incidentally at autopsy.
Recent studies have confirmed previous reports of familial clustering of BAV and left ventricular outflow tract obstruction malformations, and their association with other cardiovascular malformations.80 For example, mutations in the signaling and transcriptional regulator NOTCH1 caused a spectrum of developmental aortic valve abnormalities and severe calcification in two families with nonsyndromic familial aortic valve disease.81
Calcific deposits also can develop in the ring (annulus) of the mitral valve of elderly individuals, especially women. Although generally asymptomatic, the calcific nodules may lead to regurgitation by interference with systolic contraction of the mitral valve ring or, very rarely, stenosis by impairing mobility of the mitral leaflets during opening. Occasionally, the calcium deposits may penetrate sufficiently deeply to impinge on the atrioventricular conduction system to produce arrhythmias (and rarely sudden death). Patients with mitral annular calcification have an increased risk of stroke, and the calcific nodules, especially if ulcerated, can be the nidus for thrombotic deposits or infective endocarditis. Mitral annular calcification can also mimic a left ventricular neoplasm.
Rheumatic fever is an acute, often recurrent, inflammatory disease that generally follows a pharyngeal infection with group A beta-hemolytic streptococci, principally in children. In the past several decades, rheumatic fever and rheumatic heart disease have declined markedly but not disappeared in the United States and other developed countries. Evidence strongly suggests that rheumatic fever is the result of an immune response to streptococcal antigens, inciting either a cross-reaction to tissue antigens or a streptococcal-induced autoimmune reaction to normal tissue antigens.82
Chronic rheumatic heart disease most frequently affects the mitral and to a lesser extent the aortic and/or the tricuspid valves. Usually dominated by mitral stenosis,83 chronic rheumatic valve disease is characterized by fibrous or fibrocalcific thickening of leaflets and tendinous cords, and commissural and chordal fusion (see Fig. 5-15A and B). Stenosis results from leaflet and chordal fibrous thickening and commissural fusion, with or without secondary calcification. Regurgitation usually results from postinflammatory scarring-induced retraction of cords and leaflets. Combinations of lesions may yield valves that are both stenotic and regurgitant. Although considered the pathognomonic inflammatory myocardial lesions in acute rheumatic fever, Aschoff nodules are found infrequently in myocardium sampled at autopsy or at valve replacement surgery, most likely reflecting the extended interval from acute disease to critical functional impairment.
Degenerative mitral valve disease (mitral valve prolapse) causes chronic, pure, isolated mitral regurgitation by leaflet stretching and prolapse into the left atrium and occasionally cordal rupture.84 Owing to improved imaging technology and large community studies, a prevalence of mitral valve prolapse (MVP) of approximately 2% has been established. Potentially serious complications include progressive congestive heart failure, infective endocarditis, stroke, or other manifestation of thromboembolism, sudden death, or atrial fibrillation. Mitral valve prolapse is the most common indication for mitral valve repair or replacement.
In MVP, one or both mitral leaflets are enlarged, redundant, or floppy and will prolapse or balloon back into the left atrium during ventricular systole (see Fig. 5-15C). The three characteristic anatomic changes in MVP are: (1) intercordal ballooning (hooding) of the mitral leaflets or portions thereof (most frequently involving the posterior leaflet), sometimes accompanied by elongated, thinned, or ruptured cords; (2) rubbery diffuse leaflet thickening that hinders adequate coaptation and interdigitation of leaflet tissue during valve closure; and (3) annular dilation, with diameters and circumferences that may exceed 3.5 and 11.0 cm, respectively (see Fig. 5-15D). Pathologic mitral annular enlargement predominates in and may be confined to the posterior leaflet, because the anterior leaflet is firmly anchored by the fibrous tissue at the aortic valve and is far less distensible. The key microscopic change is myxomatous degeneration with attenuation or focal disruption of the fibrous layer of the valve, weakening the leaflet. Focal or diffuse thickening of the spongy layer by proteoglycan deposition gives the tissue an edematous, blue appearance on microscopy (called myxomatous by pathologists).85 Concomitant involvement of the tricuspid valve is present in some cases, and the aortic and pulmonary valves are rarely affected.
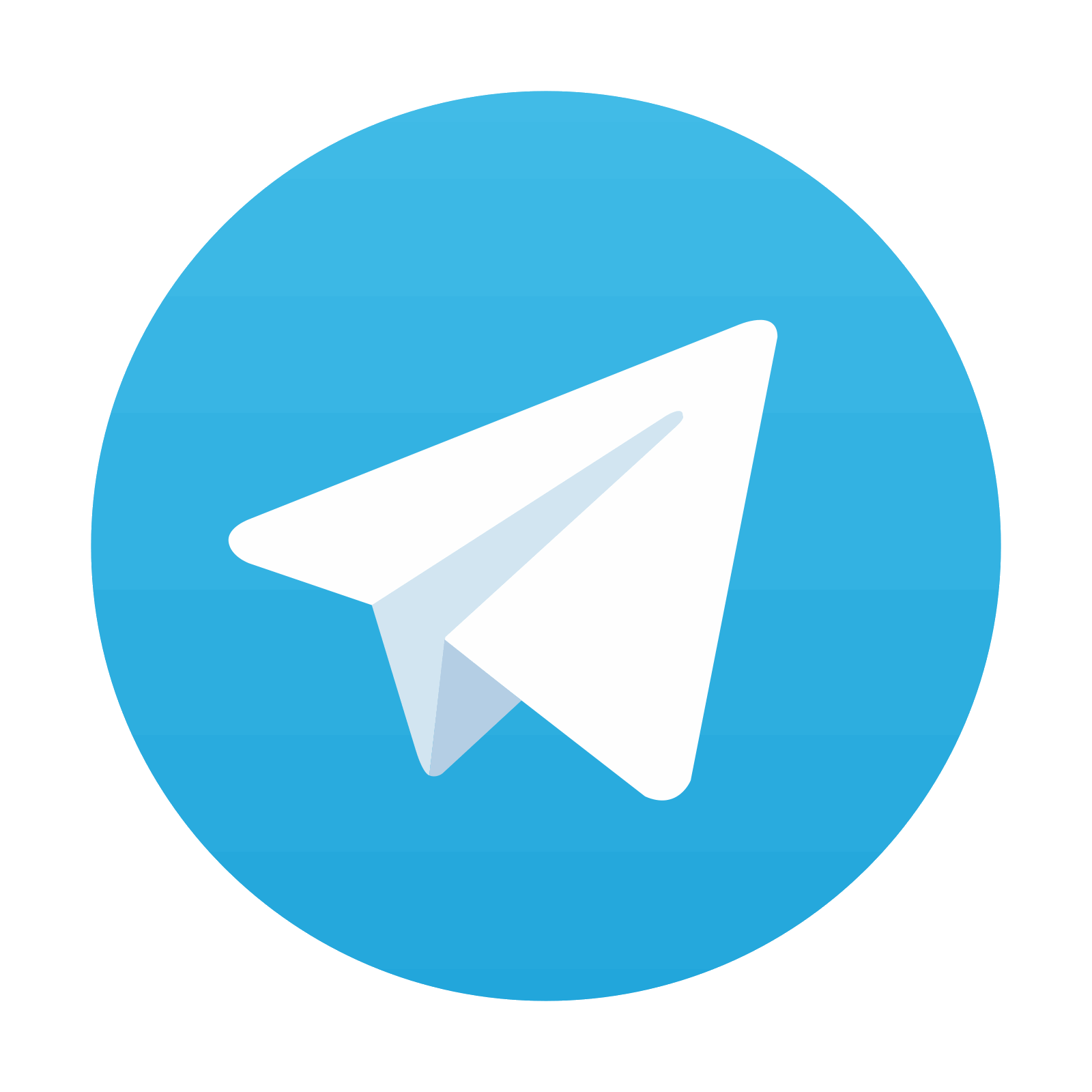
Stay updated, free articles. Join our Telegram channel
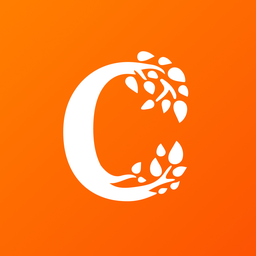
Full access? Get Clinical Tree
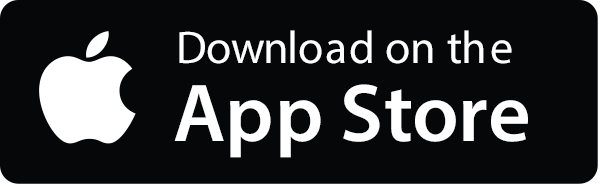
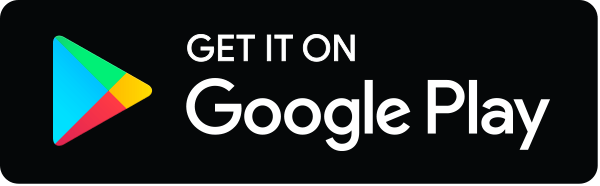