Cardiovascular Computed Tomography
Andrew O. Zurick III, MD, FACC, FASE, FSCMR
Cardiovascular Computed Tomography
For decades, investigators have sought to develop novel technologies that would allow rapid, noninvasive imaging of the heart. One such technology that has evolved rapidly in the past several decades has been cardiovascular computed tomography (CCT). Current-generation, modern CCT now permits highly detailed visualization of the coronary artery walls and lumen and provides assessment of cardiac function, valvular structures and prosthetic materials, pericardium, left atrial anatomy, congenital heart disease, pulmonary arterial and venous anatomy, and diseases of the aorta. Additionally, advancements in artificial intelligence and machine learning now permit noninvasive assessment of coronary artery physiologic function with the advent of computed tomography (CT)-based fractional flow reserve (FFR-CT).
Technology and Data Acquisition Techniques
Imaging the heart and coronary arteries with CT is an extremely technically challenging undertaking and requires sophisticated hardware and software analysis tools. Major difficulties arise because of cardiac and respiratory motion and the relatively small size of the coronary arteries, moving structures, with branches of interest in the range of 2 to 4 mm in diameter. The coronary arteries show rapid cyclic motion throughout the cardiac cycle—essentially moving in three dimensions with each heartbeat.
Over its relatively short history, several different CT scanner technologies have been used for cardiac imaging. Electron beam CT (EBCT), initially introduced in the mid-1970s, utilizes an electron source reflected onto a stationary tungsten target to generate x-rays, allowing for very rapid scan times. EBCT is well suited for cardiac imaging because of its high temporal resolution (50-100 ms) with an estimated slice thickness of 1.5 to 3 mm and the ability to scan the heart in a single breath hold. The primary use of this technique was to evaluate coronary arterial vessel wall calcium volume and density, generating a patient-specific calcium score. EBCT has since been largely supplanted by multidetector CT (MDCT) technology, which consists of a mechanically rotated x-ray source within a cylindrical gantry, with a multirow, collimated, detector located 180° opposite, that permits the simultaneous acquisition of more data (“slices”). 0.625 mm collimator rows provide for markedly increased spatial resolution and for complete acquisition of data during one breath hold. Current-generation MDCT offers improved spatial and temporal resolution, thereby making coronary CT angiography (CCTA) feasible, reproducible, and highly accurate.
CCTA was initially performed using MDCT machines capable of obtaining only four to eight slices per scan. As the technology has advanced, z-axis coverage has continued to improve; now 256-slice (and higher) scanners are available that allow acquisition of higher-resolution images without the requirement for long breath holds or extremely slow heart rates. It is currently recommended that computed tomography angiography (CTA) be performed using a minimum of a 64-slice scanner. Newer technology allows up to 320 to 512 anatomic slices to be simultaneously acquired during a single gantry rotation. With a minimal slice thickness of 0.5 to 0.625 mm, an entire heart can be imaged in a single heartbeat. Despite improved scanner hardware, most current-generation CT scanners can achieve temporal resolution approaching 140 ms but still cannot reach what can be obtained routinely in a cardiac catheterization laboratory, where fluoroscopy provides temporal resolution closer to 33 ms. To overcome the necessity of a slow heart rate, one vendor has placed two x-ray sources in the scanner gantry (so-called dual source imaging) at 90° angles to one another. This technology offers an improved temporal resolution, even with heart rates approaching 100 bpm and greater.
In the past, for coronary CTA using a single x-ray source scanner, it was typically necessary to obtain images with heart rates less than 65 bpm. Most commonly, an oral or intravenous β-blocker is given to slow the heart rate. Newer-generation scanners have somewhat lessened these rigid low heart rate requirements, although the adage still holds when imaging the heart that slower tends to be better. Coronary CTA requires intravenous administration of a contrast agent to opacify the lumen of the coronary arteries. The intravenous contrast agents used for CTA carry the same dose-dependent risks in patients with renal dysfunction as contrast agents used for cardiac catheterization, as well as the risk of an allergic reaction to iodine. Respiratory motion is minimized by patient breath holds up to 10 seconds, depending on scanner generation and patient body size. The most common data acquisition protocol utilizes a spiral mode involving continuous data acquisition during constant rotation of the x-ray tube inside the gantry, while the patient is advanced on the table through the scanner. To minimize radiation exposure, data acquisitions can be performed in sequential mode (“step and shoot”). This involves acquisition of single transaxial slices, sequentially, as a patient is advanced incrementally through the scanner.
Excessive cardiac motion can lead to blurring of the contours of the coronary vessels. For this reason, a regular heart rate is necessary for optimal imaging of the coronary arteries. Relative contraindications to performing CTA include the presence of frequent ectopic beats or atrial fibrillation. Coordinating data acquisition with the cardiac cycle involves either prospective electrocardiogram (ECG)-triggering or retrospective ECG-gating. In prospective triggering, data are acquired in a specific portion of the cardiac cycle, typically late diastole, based on simultaneous ECG recordings. In retrospective gating, data are collected during the entire cardiac cycle. Postprocessing then allows only data from specific periods of the cardiac cycle to be used for image reconstruction, as is needed.
Clinical Indications
Coronary Artery Calcium Score
Coronary artery calcium (CAC) has emerged as a robust marker of subclinical atherosclerosis and a powerful prognostic tool. CAC scoring utilizes no contrast and readily detects calcium because of its high x-ray attenuation coefficient (or CT number) measured in Hounsfield units (HU) (Figure 17.1). The Agatston scoring system assigns a calcium score based on maximal CT number and the area of calcium deposits.1 More recently, analysis of several large clinical datasets has confirmed that the coronary artery calcium score is a robust predictor of coronary events, particularly in the asymptomatic patient population, independent of traditional risk factors.2,3 In at least one study, calcium score was more predictive than C-reactive protein and standard risk factors for predicting coronary artery disease (CAD) events.4
The coronary calcium score is derived by identifying coronary arterial tree segments that have attenuation characteristics (HU) greater than a certain value (100-130 depending on software and patient size) that correlate with the attenuation due to calcium. These calcified lesions are scored by size and density with a weighting factor for increasing density. Discrete lesions are scored separately, and the density of calcium within each lesion is graded from one to four according to the HU. The sums of all the lesions are totaled to arrive at a single coronary calcium score. In general, the higher the score, the greater the amount of calcified plaque within the arterial tree. There is a positive correlation of cardiac events with this score. It is important to remember, however, that exclusively noncalcified coronary artery plaques have been reported in up to 4% of asymptomatic patients.2
The Multiethnic Study of Atherosclerosis (MESA) group published a series of articles suggesting that the calcium score is an independent risk factor for cardiac events.5 Also, MESA’s website (https://www.mesa-nhlbi.org/calcium/input.aspx) has the capacity to allow comparison of an individual patient’s calcium score against their large database. This score takes into account age, sex, and race and generates a percentile compared with the database studies. The presence of a high calcium score may prompt clinicians to use more aggressive therapy as if they were reclassified in a higher risk group or to convince patients who are reluctant to take drugs such as statins to take their disease more seriously. Recent studies have shown that a zero coronary artery calcium score demonstrated an annual event rate in asymptomatic subjects of only 0.11% (10-year risk of only 1.1%).2 Among asymptomatic patients, the incidence of abnormal nuclear stress testing is 1.3%, 11.3%, and 35.2% for calcium scores <100, 101 to 400, and >400, respectively.6 Studies of serial calcium scanning have noted that calcified plaque progression is significantly and independently associated with a worse overall prognosis.2
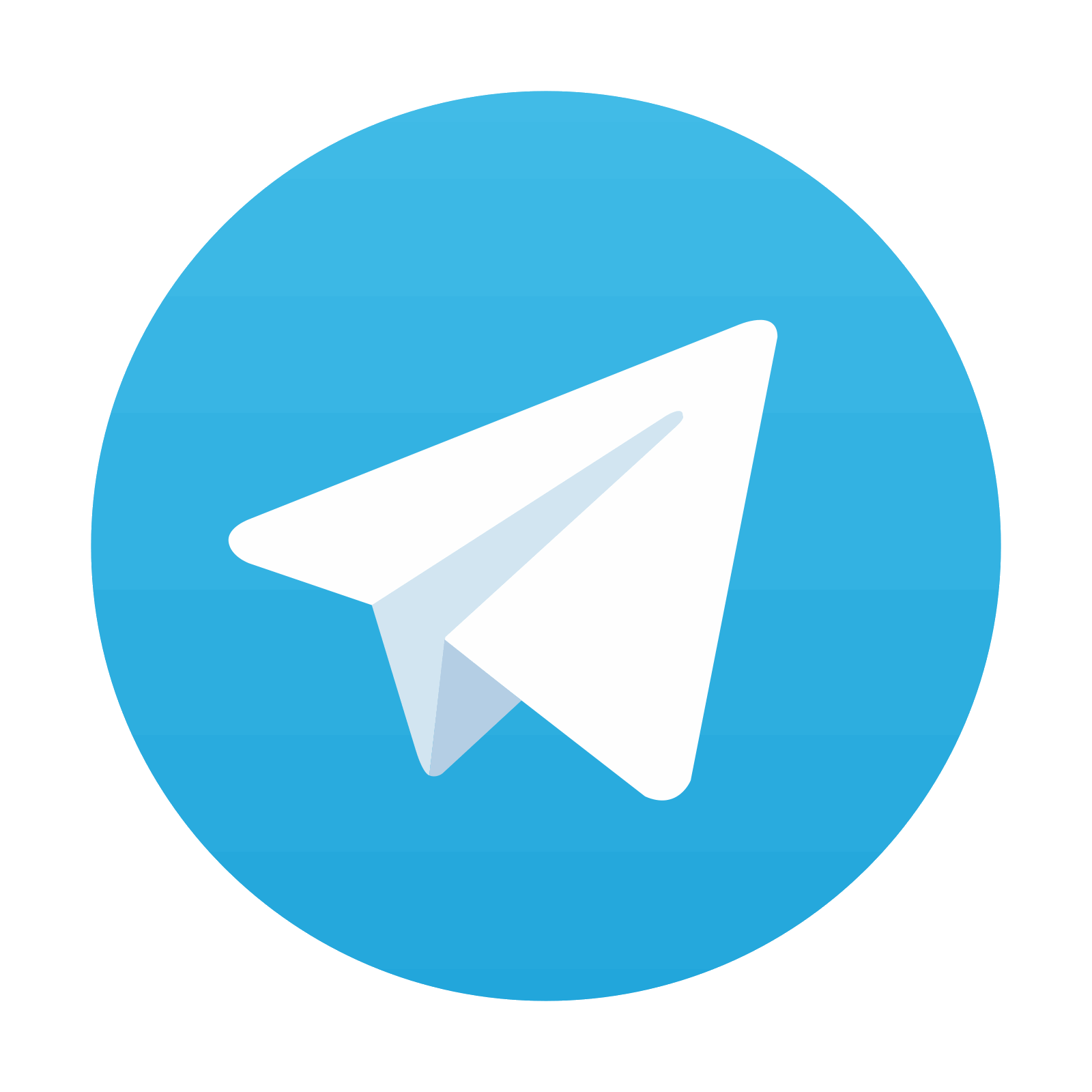
Stay updated, free articles. Join our Telegram channel
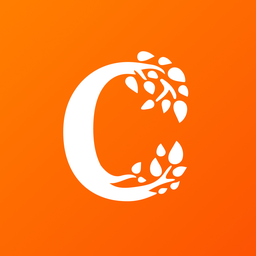
Full access? Get Clinical Tree
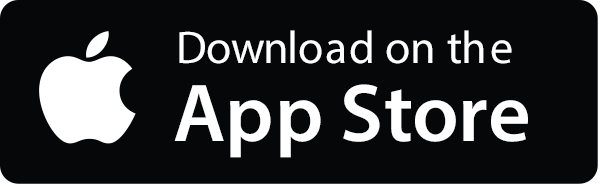
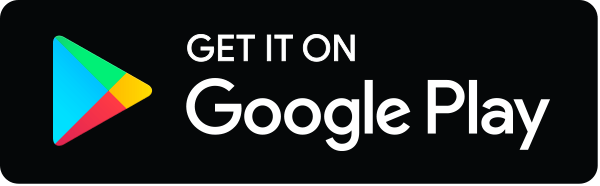
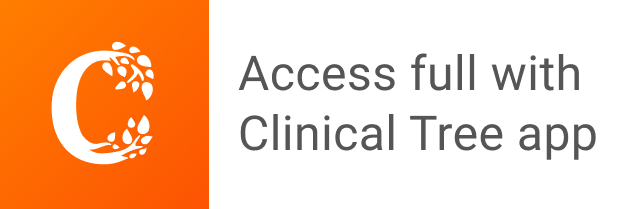