Fig. 23.1
Selected molecular imaging targets in atherosclerosis. (a) PET-CT imaging of adhesion molecule expression in apoE/mice with the 18F -VCAM–1 ligand. (b) Gd-loaded microvesicles targeted to oxLDL macrophage receptor, result in increased T1 MRI contrast. (c) Macrophage quantitation in atherosclerotic plaque using iron oxide nanoparticles that increase T2* contrast on MRI. (d) FDG/PET-CT imaging of metabolic activity in a patient with coronary heart disease using the glucose analog 18F-FDG. (e) Fluorescence Tomography-CT imaging of cathepsin protease activity in the root of apoE/mice. (f) MRI of myeloperoxidase activity in a rabbit model of atherosclerosis. (g) PET-CT imaging of integrins using 18F-Galacto-RGD in LDLr/mice. (h) MRI of fibrin within coronary artery clots in a swine model (Adapted and reproduced from Leuschner and Nahrendorf [113], [3] by permission)
Table 23.1
Non-invasive molecular imaging in cardiovascular translational research (from bench to bedside); A. Atherosclerosis, B. Ischemic heart disease, C. Ischemic injury and post–infarction remodeling, D. Heart failure
Imaging targets | Endothelial dysfunction/adhesion molecules (VCAM-1, selectins, MCP-1) | Inflammation/activated macrophages | Spotty calcifications | Apoptosis (phosphatidylserine) | MMPs | Angiogenesis (integrin αvβ3) | Thrombus (fibrin, glycoprotein IIb/IIIa) | |
---|---|---|---|---|---|---|---|---|
A. Atherosclerosis | Pre-clinical | PET, SPECT, MRI, optical imaging, ECHO. | PET, SPECT, MRI, CT | Optical imaging | SPECT, PET, MRI | SPECT, MRI, optical imaging | PET, MRI, optical imaging, ECHO | MRI, PET, ECHO |
Clinical | No | PET/FDG (clinical trials) MRI (studies) | PET/NaF (initial studies) | Limited data | No | Limited data | Fibrin-targeted MRI (clinical trials) |
Imaging targets | Myocardial perfusion | Ischemic memory | |
---|---|---|---|
B. Ischemic heart disease | Pre-clinical | SPECT and PET MPI, CE-MRI, ECHO, CT | SPECT/BMIPP, ECHO-microbubbles |
Clinical | SPECT and PET MPI, CE-MRI, ECHO, CT | SPECT/BMIPP |
Imaging targets | Myocardial perfusion | Apoptosis/necrosis | Inflammation/activated macrophages | MMPs | RAAS | Angiogenesis/integrin αvβ3, VEGF | |
---|---|---|---|---|---|---|---|
C. Ischemic injury and post-infarction remodeling | Pre-clinical | SPECT and PET MPI, CE-MRI, ECHO, CT | Dual-contrast MRI | PET/FDG, MRI, ECHO-microbubbles | SPECT, optical imaging | SPECT, PET | PET, SPECT, MRI, ECHO-microbubbles |
Clinical | SPECT and PET MPI, LGE-MRI, ECHO, CT | Limited data on apoptosis/necrosis | Limited data | No | Limited data | Limited data |
Imaging targets | Myocardial perfusion | Myocardial viability | Myocardial innervation | |
---|---|---|---|---|
D. Heart failure | Pre-clinical | SPECT and PET MPI, CE-MRI, ECHO, CT | Glucose metabolism (FDG PET) | PET (HED, EPI), SPECT (MIBG) |
FA metabolism (SPECT, PET) | ||||
Oxidative metabolism (PET) | ||||
Cell membrane integrity (TL-SPECT) | ||||
Intact mitochondria (Tc-SPECT) | ||||
(Recruitment of) myocardial thickness/motion (ECHO, cine MRI, G-SPECT) | ||||
LGE-MRI | ||||
Clinical | SPECT and PET MPI, CE-MRI | The same as in pre-clinical imaging | PET (HED), SPECT (MIBG) |
23.2.1.1 Preclinical Molecular Imaging of Atherosclerosis and the Vulnerable Plaque
Endothelial dysfunction is one of the first changes occurring in patients with ATH. Imaging of EC activation has been accomplished targeting VCAM-1 in mice models with ATH, using microPET/CT imaging with the 18F -VCAM–1 ligand or with small iron-oxide nanoparticles (SPIOs) [VCAM-1 targeted USPIO derivative (R832)] for microMRI imaging, offering also the possibility to add fluorescent dyes for optical verification in vivo [3]. Microbubbles that target VCAM-1, and P-selectin for molecular ultrasound (US) imaging have also been developed [4]. In addition, studies in rabbits have confirmed monocyte trafficking in atheroma using the monocyte chemotactic protein-1 (MCP-1) labeled with 99mTc (microSPECT) [5]. All of these agents remain still in the preclinical level and further studies will be required to test their effectiveness towards clinical applications.
INFL is a key feature of plaque destabilization and therefore an attractive imaging target. A number of techniques and agents have been developed. Herein we describe these which have already been introduced in the clinical setting and those which have shown promising results at a preclinical level: Activated macrophages have been targeted with radionuclide methods based either on their increased glycolytic activity (by FDG PET) or by targeting specific receptors. The feasibility of plaque INFL imaging with 18F- Fluoro-deoxyglucose (FDG) was established in animal (small and large) models of aortic ATH, with results showing a three- to fivefold greater 18F-FDG uptake in atherosclerotic aortas than in normal aortas [6]. Several receptors upregulated in activated macrophages have been exploited in pre-clinical imaging of ATH, such as LOX-1 with the use of 99mTc anti-LOX-1 antibody for SPECT imaging [7] or peripheral benzodiazepine receptor (PBR) with the use of 11C-PK11195 for PET imaging in mice and rabbits [8]. An MRI based approach has also been tested in animal models (rabbits) for imaging of vessel wall INFL using SPIOs [9] or alternatively with the Gd-containing immunomicelles [10] or lipid-based nanoparticles [11]. In addition, MDCT has been employed in imaging of INFL with the use of iodinated [12] or gold [13] nanoparticles that selectively accumulate into macrophages. Although promising, these results remain yet to be confirmed in clinical studies.
Arterial calcification is part of the development of ATH and is related to cardiovascular risk. Active calcification progressively increases given that the inflammatory cascade contributes to calcium deposition. Although large calcifications inside the plaque have been linked by some to plaque stability, spotty calcifications, especially inside the fibrous cap, are suggested to cause plaque rupture and thrombotic events, as it is assumed to be a product of osteogenic action by osteocytic- and chondrocytic-like cells inside the plaque [14]. In a mouse model of ATH, Aikawa et al. [15] were able to detect INFL and microcalcifications in early atherosclerotic plaques using a nanoparticle-based contrast agent to visualize macrophages and a bisphosphonate-derivatized near-infrared fluorescent imaging agent to detect calcifications. They showed that macrophage infiltration in early atherosclerotic plaques precedes calcification.
Another feature of plaque instability is the high rate of macrophage APO. It is a typical feature of fibrous caps of vulnerable and ruptured atherosclerotic plaques and it has also been an imaging target by a variety of non invasive techniques. In a pioneering study of molecular imaging of APO with 99mTc-labeled annexin-V (99mTc-anxA5) SPECT, Kolodgie et al. [16] demonstrated the feasibility to target APO in rabbit models of ATH. Further experimental studies in rabbits have demonstrated that novel therapies with caspase inhibitors suppress atherosclerotic plaque APO in vivo using 99mTc-anxA5 microSPECT/CT [17]. Moreover, in a recent study in mice models using novel radiotracers to image APO (99mTc-HIS-AnxA5) and N (124I hypericin) with SPECT/CT and PET/CT respectively, De Saint-Hubert et al. [18] showed that APO and N imaging is feasible in principle, but there are still hurdles to overcome including further optimization in biodistribution probes characteristics that is essential before such findings are translated to humans. APO -targeted ultra small super paramagnetic iron oxide derivatives (USPIO-R826) have also been tested with MRI in mice models and seem to be highly promising tools for ATH imaging contributing to the detection of vulnerable plaques [3].
With vessel REM that occurs in ATH, there is activation of several proteases (e.g., MMPs or cathepsin G) that also have significant detrimental effects on cap thickness and therefore plaque stability. The advantage of imaging MMPs is the assessment of the activity of macrophages rather than their density in vulnerable plaques. Up to date, 23 MMPs have been identified. A large variety of synthetic MMPs ligands with high affinity has been developed in the past. These small molecules act as MMP inhibitors and were first tested as anticancer drugs. Different MMPs imaging approaches for SPECT, MRI and optical imaging have been successfully tested in various animal models of ATH. Recently the first GMP-conform MMP inhibitor labeled with 18F suitable for PET imaging was described [19], paving the way for the first studies in humans in the near future.
An additional imaging target is intraplaque angiogenesis that is manifested by proliferation of medial vasa vasorum and has been implicated in rapid plaque growth, intraplaque haemorrhage, and plaque rupture. Integrin αvβ3 is a potential imaging target for plaque and vasa vasorum neovascularization. Winter et al. [20] showed in rabbits that in vivo imaging of neovascularization of atherosclerotic plaques using MRI targeting αvβ3 paramagnetic nanoparticles is feasible and might provide a method for defining the burden and evolution of ATH. More recently, cyclic peptides that contain the Arg-Gly-Asp (RGD) attachment site labeled for optical imaging or PET have demonstrated focally increased uptake in advanced, macrophage-rich atherosclerotic lesions of hypercholesterolaemic mice [21].
Thrombus formation may also be a useful target for identification of complicated atherosclerotic lesions as well as sources of thrombo-embolism. Inflamed endothelium and cells participating in the development of the necrotic core show high levels of fibrin and thrombin expression. Molecular MRI with the fibrin-targeted contrast-agent EP-2104R has been demonstrated in animal models allowing selective visualization of acute coronary, brachiocephalic artery, cardiac, and pulmonary thrombi [22]. MRI of thrombus has been otherwise achieved at high fields (9.4 T) in mouse models of carotid thrombosis by use of single-chain antibody-conjugated SPIOs targeted to the glycoprotein IIb/IIIa of activated platelets [23]. This approach represents a novel noninvasive technique allowing the detection and quantification of platelet-containing thrombi and could be further tested in humans. Whether it can also be applicable to assessing thrombosis in human coronary vessels where plaque assessment is challenging due to the small vessel size remains unknown at present. US microbubble targeting of the thrombus has also been investigated using an approach based on the surface conjugation of ligands that recognize the platelet glycoprotein IIb/IIIa, fibrin, and tissue factor. In terms of radionuclide imaging, a recently described tracer (99mTc-fucoidan) has demonstrated its use to image P-selectin overexpression in arterial thrombosis and EC activation in animal models [24]. Three new fibrin-targeted PET probes (FBP1, FBP2, FBP3) have been tested with micro-PET/MRI in rat models of carotid artery thrombosis with promising results, as identification of thrombi was confirmed in those experimental studies ex vivo by autoradiography [25].
23.2.1.2 Clinical Molecular Imaging of Atherosclerosis and the Vulnerable Plaque
A decade ago FDG uptake was for the first time described as an incidental finding in the vascular wall of large arteries in oncological patients. Yun et al. [26] reported that along all vascular beds (aorta, iliac, and femoral arteries), age and hypercholesterolemia were correlated with the degree of FDG uptake. The clinical feasibility of 18F-FDG plaque imaging has been demonstrated in patients with carotid, aortic, femoral, and iliac ATH [27–29] with proven high reproducibility [27] (Fig. 23.2). Positive correlation has been observed between 18F-FDG PET activity and CD68-immunostaining for macrophages in endarterectomy samples from carotid plaques [29], as well as between FDG and increased biomarkers of angiogenesis [vascular endothelial growth factor (VEGF)] [30]. Although quantification of FDG uptake is a highly reproducible measure, it does vary over time suggesting that INFL may be a transient feature of ATH which waxes and wanes as disease progresses [30].
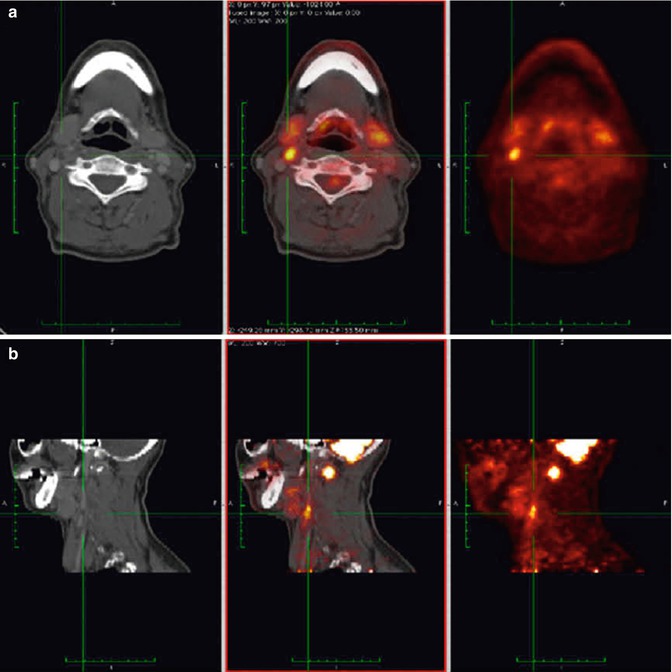
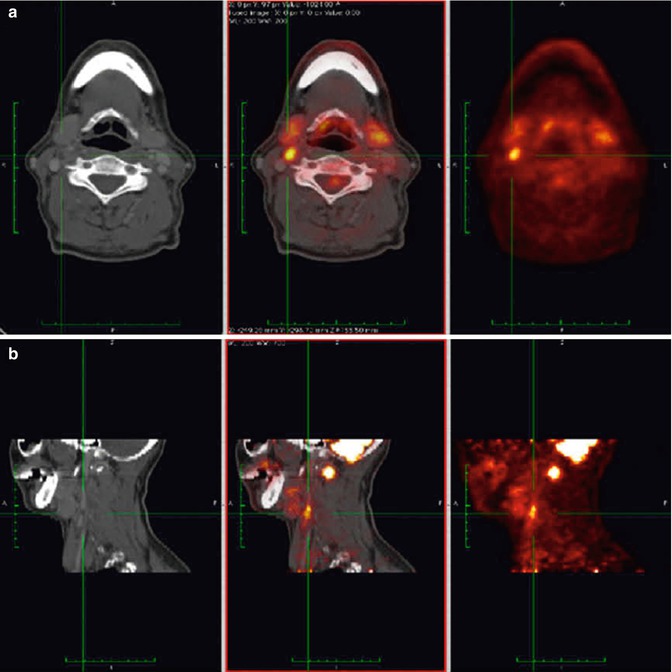
Fig. 23.2
Example of qualitative assessment of FDG uptake in right-side carotid artery plaque from a patient with high plaque uptake. (a) Transverse and (b) sagittal slice of same patient. Both CT (left), FDG-PET (right) and fused image (middle) are shown (Adapted and reproduced from Graebe et al. [114], [29] by permission)
Clinical FDG PET/CT studies have shown that vascular calcification (as assessed by CT) and vascular metabolic activity (assessed by PET) rarely overlap, whereas plaque calcification was inversely related to PET and histologic biomarkers of INFL, suggesting that these findings represent different stages in the evolution of atheroma [31]. INFL burden assessed by PET corresponds to high risk morphology plaques (positive REM and low attenuation), as assessed by CT [32]. The time interval between symptoms [transient ischaemic attack (TIA)/stroke] and a significantly positive ipsilateral FDG PET result in the culprit lesion was also investigated and found to be about 38 days [33]. The observation that PET imaging in close proximity to the time of the event (TIA or stroke) reveals higher lesional-to contralesional max SUV ratios in the culprit vessels compared to those with chronic stenosis was also confirmed more recently by a different research group [34]. Whether FDG PET could improve the clinical management of patients with carotid stenosis remains to be proven, but at the moment, FDG-PET can only complement conventional anatomic imaging (US, CT and MRA) towards risk assessment for future stroke [34]. The interrelationship of atherosclerotic disease across arterial beds has also been evaluated using FDG imaging. Rudd et al. [31] have demonstrated a positive relationship between uptake in adjacent territories and along paired left and right arterial beds. Multi-vascular evaluation of disease has been proposed, given that high FDG uptake across major vascular beds including the aorta, iliac, and carotid arteries is a predictor of future cardiovascular events [35] (Fig. 23.3).
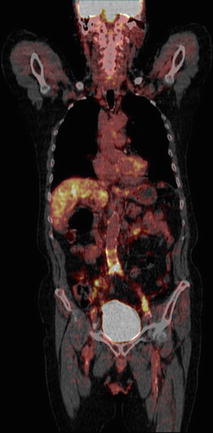
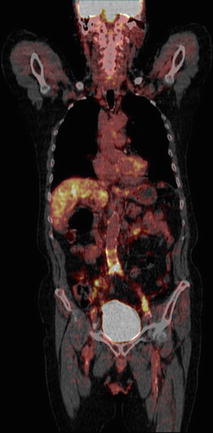
Fig. 23.3
Coronal 18F PET/CT image of a patient depicting high uptake of tracer in the lower part of the abdominal aorta and the left common iliac artery suggestive of atherosclerosis
Carotid FDG-PET/CT imaging has been employed for the first time in a phase 2b, double-blind, multicentre study (dal-PLAQUE), as a co-primary endpoint together with MRI-assessed indices to study the drug dalcetrapib that modulates cholesteryl ester transfer protein (CETP) activity to raise high-density lipoprotein (HDL) cholesterol. Total vessel area that was used to assess ATH burden by MRI was reduced in patients given dalcetrapib. However, PET/CT showed no evidence of change of vascular INFL assessed by FDG [36]. In a recent study, statin therapy (atorvastatin) produced significant rapid dose-dependent (10 versus 80 mg) reductions in FDG uptake within the artery wall, that may represent changes in atherosclerotic plaque INFL indicating a possible role of FDG PET in detecting early treatment effects in patients at risk or with established ATH [37].
Despite favorable results from a number of clinical studies, it should be noted that FDG signal is not only determined by inflammatory macrophages but other parameters; for instance, thrombus activity or SMCs function, which can also affect tracer uptake. More specific targets have also been tested clinically by Gaemperli et al. [38] demonstrating non-invasive detection and quantification of intraplaque INFL with peripheral benzodiazepine receptor PBR PET/CT imaging (11C-PK11195). Other surrogate markers of atheroma INFL have also been used in humans including 68Ga-DOTATATE and 11C-choline. 68Ga-DOTATATE binds to somatostatin receptors subtype 2 that are expressed by macrophages [39]. 11C-choline differs in that it is taken up by inflammatory cells—primarily macrophages, following which it undergoes phosphorylation and is metabolized into forming phosphatidylcholine that is eventually incorporated into the cellular membrane [40].
Beyond imaging of larger arterial beds, which has experienced rapid clinical translation, molecular imaging of coronary arterial plaques has also made progress recently (Fig. 23.4).18F-FDG has been tested in this setting by various groups but with limited success, since physiological myocardial uptake of this tracer posses difficulties in identifying local INFL in coronary lesions in addition to those related to motion artifact, and partial volume effect. Employing a high-fat, low-carbohydrate diet before FDG-PET imaging to lower the physiological glucose uptake in the myocardium, studies have demonstrated the feasibility of FDG uptake in coronary plaques. Rogers et al. [41] studied FDG uptake in the left main coronary artery, as this is larger, more remote from myocardium, and less mobile. They found greater FDG uptake in left main artery in patients with acute coronary syndromes (ACS) compared to those with stable angina [41]. Dweck et al. [42] have employed PET/CT imaging with 18F-sodium fluoride (18F-NaF), a radiopharmaceutical used for skeletal imaging which provides relevant information regarding active micro-deposition of calcium in atherosclerotic plaques, a process that is associated with plaque instability. They found that 18F-NaF uptake was higher in patients with coronary ATH versus control subjects and correlated with the calcium score, although 40 % of those with scores >1,000 displayed normal uptake [42]. Patients with increased coronary 18F-NaF activity had higher rates of prior cardiovascular events and angina and higher Framingham risk scores [42] (Fig. 23.5). More recently, the same group have reported that 18F-NaF localizes more avidly in plaques of patients with symptomatic carotid disease and in ruptured plaques of patients with acute MI compared to patients with stable coronary artery disease (CAD) [43]. This technique holds great promise for identifying high-risk and ruptured plaque however, further studies are needed to establish whether it can improve management of patients with CAD.
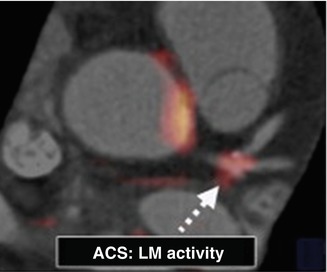
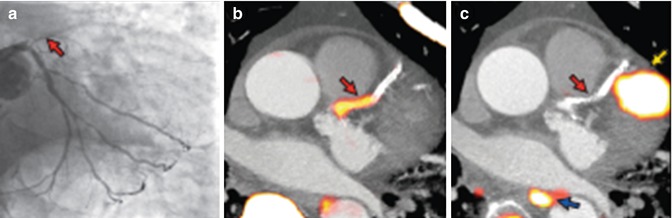
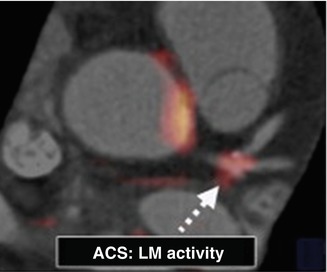
Fig. 23.4
Coregistered FDG PET/CT images showing FDG uptake at the trifurcation of the LM in a subject presenting with ACS. ACS acute coronary syndrome, CT computed tomography, FDG fluorodeoxyglucose, LM left main coronary artery, PET positron emission tomography (Adapted and reproduced from Rogers et al. [41], by permission)
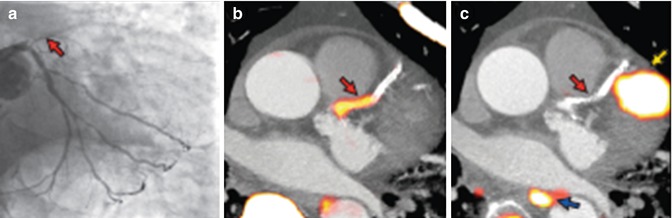
Fig. 23.5
Focal 18F-NaF and 18F-FDG uptake in patients with myocardial infarction and stable angina. Patient with acute ST-segment elevation myocardial infarction with (a) proximal occlusion (red arrow) of the left anterior descending artery on invasive coronary angiography and (b) intense focal 18F-NaF uptake (yellow–red) at the site of the culprit plaque (red arrow) on the combined positron emission and computed tomogram (PET-CT). Corresponding 18F–FDG PET-CT image (c) showing no uptake at the site of the culprit plaque. Note the significant myocardial uptake overlapping with the coronary artery (yellow arrow) and uptake within the oesophagus (blue arrow) (Adapted and reproduced from Joshi et al. [43], by permission)
Early studies involving symptomatic patients undergoing carotid endarterectomy found that up to a 24 % change in MRI signal could be observed for plaques 24 h after SPIOs delivery with histological proof of macrophage uptake [44]. The fast iron turnover renders repetitive imaging with this technique feasible and potentially useful to monitor treatment efficacy. Indeed, a recent prospective human study, ATHEROMA (Atorvastatin Therapy: Effects on Reduction of Macrophage Activity), showed the feasibility of using SPIOs with MRI to monitor serially the effect of atorvastatin on plaque INFL at 6-week intervals [45]. Reduction of SPIOs uptake was detected as early as 6 weeks after high-dose (80 mg) atorvastatin therapy, months to years earlier than changes in plaque morphology. These findings reaffirmed the advantage of molecular imaging over morphometric imaging for assessment of the effectiveness of plaque-altering therapy.
Neovascularization in carotid plaques has been assessed semi-quantitatively by contrast-enhanced ultrasonography (CEUS) and it was shown that significant acoustic plaque enhancement is correlated both with histopathology and clinical presentation. This approach takes advantage of the fact that US contrast agents are pure intravascular tracers and hence, CEUS allows for the assessment of the amount of blood contained in the microvasculature within the region of interest. US molecular imaging, with specific ligands, such as for instance monoclonal antibodies, targeting integrins such as αvβ3, remain for the time being in the domain of preclinical research. Angiogenesis imaging with 18F-Galacto-RGD PET/CT could be another option, with recent data from 10 patients with high-grade carotid artery stenosis scheduled for carotid endarterectomy, demonstrating specific tracer accumulation in atherosclerotic carotid plaques, which correlates with immunohistochemistry of avb3 expression [46].
Regarding APO imaging, 99mTc-anxA5 has been tested with SPECT in a small pilot study of four patients for its ability to detect cellular APO associated with unstable human carotid plaques [47]. Significant 99mTc-anxA5 uptake was found in the culprit lesions of patients with a recent history of transient ischemic attack, concordant to ex vivo histological confirmation after endarterectomy. Future prospective outcome studies in a larger group of patients will help determine its true discriminatory potential.
For thrombus imaging fibrin-targeted contrast agent EP-2104R has been used in a phase II clinical trial that included 11 patients with recent thrombosis (intracardiac, thoracic aorta, carotid artery) indicating that the agent provides strong enough signals for visualization of intracardiac, extracardiac arterial, and venous thrombi [48]. Black-blood MRI was performed 3.5 h after agent injection showing very good thrombus-to-adjacent soft tissue contrast and a sensitivity of 84 % for detection of known thrombi in the aforementioned vascular territories with this agent [48]. Ongoing efforts aim at increasing further its imaging sensitivity by optimizing the contrast dose and imaging parameters. Although the relatively long administration-to-imaging time possibly precludes widespread application of EP-2104R for acute arterial thrombosis syndromes, fibrin MRI may, at least conceptually, offer an alternative option to transesophageal echocardiography (ECHO) for left atrial thrombi. In addition, detection of subclinical thrombi on carotid atheroma could identify high risk patients.
In summary, significant progress has been made recently in the clinical setting towards identification of plaques which are prone to rupture. These first steps are important for the clinical community, as identification and appropriate treatment of these high-risk plaques can result in their stabilization and potentially reduce the incidence of stroke, acute MI and sudden cardiac death. It should be emphasized that we still need data on the natural history of these plaques, and at present, we lack outcome data (in terms of soft and hard cardiovascular events) from well designed prospective randomized trials upon which to develop treatment criteria for these plaques.
23.2.1.3 Imaging of Morphological Plaque Changes: Lipid Core and Fibrous Cup Formation
MDCT allows imaging of the vessel wall, potentially providing insights into the characteristics and extent of intramural ATH. Three parameters have been shown to be related to cardiovascular events: (1) CT density (<30 HU is an indicator of vulnerability); (2) lumen irregularities and (3) positive REM index. More recently, such parameters have been tested in a study aiming to assess their value for predicting ACS. Semiautomatic plaque quantification was employed (reporting plaque volume, burden area, noncalcified percentage, attenuation and positive REM). In this study, 1,650 patients were included and ROC analysis was performed comparing a model incorporating Framingham risk score (FRS) and conventional cardiac computed tomography angiography reading (consisting of calcium score, luminal stenosis, extent of CAD, and morphology assessment) with a model that also included semiautomated plaque quantification. The areas under the curve were 0.64 and 0.79 respectively, (p < 0.05), indicating an enhanced ability of CT to detect potentially vulnerable plaque by providing additional information beyond that obtained from conventional reading [49].
MRI has also been tested for assessing atheromatous plaque morphology particularly of large or “static” arteries, such as the carotid arteries. Lipid and fibrotic plaque components have been accurately quantified on T2-weighted images, in both animals and humans. T2-weighted images have also been used to measure the fibrous cap thickness and to identify fibrous cap rupture and intraplaque hemorrhage, features that are commonly found in symptomatic carotid ATH [50]. The main limitation of MRI in identifying vulnerable plaques is its relatively poor reproducibility. To overcome this limitation newer techniques have been developed and applied in patients. These include diffusion weighted, gado-fluorine enhanced and time of flight imaging which have the potential to identify lipid-rich necrotic core and fibrous cap as well as intra-plaque hemorrhage, an indicator of plaque rupture. Attempts have also been made for plaque assessment in coronary arteries. In a recent prospective study by Noguchi et al. [51], 568 patients with suspected or known CAD underwent non-contrast T1-weighted imaging to determine whether coronary high-intensity plaques (HIPs), which are associated with characteristics of vulnerability can predict future coronary events. The results showed that HIPs are significantly associated with coronary events, and may thus represent a novel predictive factor.
23.2.2 Ischemic Heart Disease
The most frequent cause of myocardial ischemia in man is coronary ATH. This can lead to chronic ischemic syndromes or to acute episodes of plaque instability that can be manifested as acute myocardial infarction (MI) or sudden death. In the clinical setting, non-invasive assessment of ischemic heart disease (IHD) focuses on functional and metabolic effects of coronary flow limitation related to stenotic plaques. The heart is metabolically a very active organ and requires a high level of oxygen delivery. Furthermore oxygen extraction from delivered blood is high even at rest. Any increase in oxygen demand therefore must be met by increasing coronary and microcirculatory blood flow, as there is little scope to increase oxygen extraction. The balance between myocardial oxygen requirements and oxygen delivery is central to understanding the mechanisms by which ischemia can occur. On a metabolic level, the myocardium uses a variety of substrates, but under physiological conditions in the fasting state most of the energy required comes from the oxidation of fatty acids (FA) and to a lesser degree from glucose. When glucose or insulin levels are high, such as after a meal, glucose oxidation increases and fatty acid use is suppressed. Myocardial ischemia results in a metabolic shift from FA to glucose, which results in 11 % more ATP produced per molecule of oxygen [52].
As myocardial oxygen demand increases or coronary blood flow falls, autoregulatory and metabolic regulatory mechanisms are activated towards maintaining myocardial perfusion at a normal level. When these compensatory changes (including autoregulation and metabolic regulation) become exhausted, ischemia finally results as myocardial blood flow falls. In patients with atherosclerotic heart disease, compensatory mechanisms to maintain myocardial perfusion in the face of significant epicardial stenoses are already active. Vasodilatation of the distal vascular bed occurs and pressure gradient across the stenosis increases. This exhaustion of compensatory mechanisms in patients with CAD is also compounded by abnormal endothelial function and impaired endothelium-derived vasodilator mechanisms. The first abnormality to become apparent during ischemia is therefore reduced perfusion to the affected territory. It is not until a diameter stenosis of around 80 % that resting perfusion is critically reduced, and as a rule of thumb, any stenosis less than 40 % diameter is unlikely to have any haemodynamic consequences even during maximum coronary dilatation. The pathophysiology of myocardial ischemia involves a series of progressive changes that have been described in terms of a ‘cascade’ from the cellular level through perfusion abnormalities, contractile dysfunction, electrocardiographic abnormalities and finally symptoms [53].
23.2.2.1 Functional and Metabolic Imaging in Ischemic Heart Disease
In patients with stenotic plaques but no reduction in flow at rest due to compensatory mechanisms, perfusion abnormalities can be induced by stressing the perfusion system (Table 23.1B). Rest and stress radionuclide myocardial perfusion imaging (MPI) is a well established technique for diagnosis and risk stratification. Comparison of tracer distribution at rest and exercise or pharmacological stress can describe the presence, severity, and extent of myocardial ischemia caused by flow-limiting coronary stenoses. There are alternative well validated techniques for non-invasive assessment of myocardial perfusion and these include ECHO and MRI, for an in depth review of the subject and comparisons of diagnostic accuracy that is beyond the scope of this chapter, the reader is referred to selected references [54, 55].
A different approach is to image the metabolic and molecular sequels of myocardial ischemia. This is termed “ischemic memory” and may reveal the ischemic origin of recent chest pain and define the extent of myocardium compromised by ischemia (Table 23.1B). There are experimental and clinical data demonstrating the feasibility and significance of metabolic radionuclide imaging in patients with stable disease but also in those with suspected ACS mostly using radiolabeled FA such as 123I-BMIPP SPECT [56, 57]. However, despite encouraging results, a number of issues remain unsettled, including optimal imaging protocols, widespread tracer availability, utility of metabolic imaging in diabetic patients, incremental value for diagnosis and prognosis over and above that obtained by perfusion data and impact of radionuclide metabolic imaging on clinical management. Ischemic memory imaging with ECHO has also been achieved in mice models using microbubbles targeted to the EC adhesion molecule P-selectin, which is expressed in response to only a mild ischemic stimulus. P-selectin–targeted imaging has been shown to detect brief myocardial ischemic injury even after the ischemic insult has resolved and in the absence of MI [58] (Fig. 23.6). No clinical studies using this technique have been performed so far. For the initiation of clinical trials, several developments need to take place both for the contrast agents that are being used and the imaging technology for tracer detection.
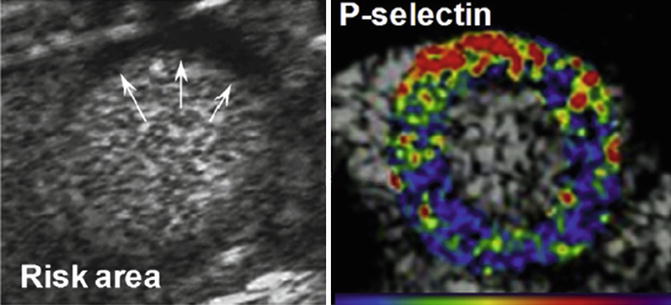
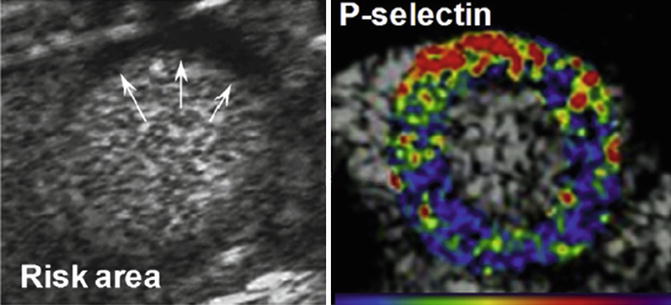
Fig. 23.6
Contrast echocardiographic imaging of recent myocardial ischemia with P-selectin–targeted microbubbles. Short-axis contrast echocardiographic images from a mouse illustrating hypoperfusion (arrows) of the anterior wall on perfusion imaging during brief occlusion of the left anterior descending (left) and enhancement within the previously ischemic anterior territory with P-selectin–targeted microbubbles injected intravenously 45 min after reflow. Color scale at bottom (Adapted and reproduced from Inaba and Lindner [115], [58] by permission)
23.2.2.2 Specific Issues Related to Ischemia-Reperfusion Injury and Post-infarction Remodeling
Acute MI is a common cause of REM of the left ventricle (LV). It is estimated that despite primary percutaneous coronary intervention (PCI) and standard current therapy, around 30 % of anterior MI will develop REM. The latter is a complex process by which mechanical, neurohormonal and genetic factors alter ventricular structure and function leading to reduced mechanical performance, electrical instability and sudden death. It is an important aspect of heart failure (HF) progression, characterized by dilation and change of the shape of the left LV and evolving alterations in the ventricular wall which include hypertrophy, loss of myocytes and increased interstitial fibrosis [59]. The three major biomechanical mechanisms contributing to the increase of LV volumes over time after MI are: (a) expansion of the infarct in the sub-acute phase, (b) subsequent non-ischemic infarct extension into the adjacent noninfarcted region, and (c) hypertrophy and dilation of non-infarcted myocardium in the chronic phase [59]. The main factors associated with REM are size of infarction, residual viability within the infarct territory, anterior location and late or unsuccessful (or absence of) reperfusion therapy both at the epicardial vessel level and at the microvasculature level (no reflow) [59].
Imaging techniques have been employed for prediction of LV REM. Non-invasive techniques in the form of ECHO, MRI, CT and radionuclide imaging have been utilized in this setting, as they are well suited for assessing parameters which either constitute phenotypic expressions of REM (e.g. alterations of heart size, shape and mass, end diastolic and end-systolic volumes and regional contractility) or are by themselves determinants of the REM process (e.g. myocardial perfusion, fibrosis and viability). ECHO which is widely available, is the most commonly used technique for prediction of LV REM, but MRI because of its high accuracy and reproducibility of measurements is currently the gold standard to assess the aforementioned parameters as well as microvascular obstruction, tissue oedema and infarct size at the early stage post-acute MI [60]. The later is a key determinant of long-term LV REM, however, microvascular obstruction, indexed by intramyocardial haemorrhage on T2-weighed images has also been found to be a powerful predictor of changes in global function and LV end-systolic volume [61]. Tomographic radionuclide imaging with 99mTc-labelled tracers (Sestamibi and Tetrofosmin) and ECG gating synchronisation (ECG-gated SPECT) is another option for predicting LV REM in the post primary PCI setting, as it offers the benefit of assessing both perfusion and function in the same myocardial segments as well as salvaged myocardium and final scar size, but it also allows accurate and highly reproducible measurements of global LV function and volumes [62].
However, there still exists a need for upstream imaging biomarkers that will allow timely and targeted intervention before disease has progressed beyond the point of no return. A key element to this, is knowledge of changes occurring at cellular or extracellular level that has been acquired over the last few years. It is now recognized that LV REM is characterized by a regression to the fetal pattern, i.e. increase of β-MHC, α-actin, ANP overexpression, sarcoendoplasmic reticulum Ca2+-ATPase activity decrease, and a shift of myocardial metabolism towards glucose utilization [59]. In the following paragraphs, we discuss selected novel imaging strategies which could complement the more conventional techniques described above and may provide new possibilities for the management of post MI patients (Table 23.1C).
23.2.2.3 Molecular Imaging Targets in Ischemic Injury and Post-infarction Remodeling
APO is different from N, as it is a reversible process, and hence a therapeutic target, whereas N is not. The dual-contrast imaging technique for high-resolution MRI with both annexin-V labelled nanoparticle (AnxCLIO-cy5.5) to image APO and delayed-enhancement imaging with a novel gadolinium chelate (Gd-DTPA-NBD), to image N, has great potential for clinical translation as demonstrated recently in a transgenic mouse model of myocardial ischaemia [63]. This method allowed the apoptotic cells to be differentiated from necrotic cells, which further helped to identify a group of apoptotic yet viable cells in the myocardium (4–6 h after ischemia reperfusion) that could benefit from antiapoptotic therapy. MRI agents that bind to exposed DNA are also under investigation in animal models [64].
Post-infarction INFL with FDG PET imaging has been assessed in mice models and in selected human case studies shortly after myocardial infarction and reperfusion in combination with LGE MRI [65, 66]. FDG uptake on PET, corresponding to matching LGE on MRI, shortly after myocardial infarction and reperfusion, indicates INFL and/or a change in myocardial metabolism (Fig. 23.7). Post-infarction INFL has also been attempted to be assessed more specifically in mice models with MRI and contrast microbubble ECHO to image monocytes/macrophages or myeloperoxidase (MPO) activity [67–70] providing considerable information regarding the progression of wound healing and scar formation in the LV after MI. In particular, in the recent study by Lee et al. [66], Gd-DTPA–enhanced infarcts showed high FDG uptake on day 5 after MI in a mouse model. Surprisingly, there was also considerable monocyte recruitment in the remote myocardium in parallel with a robust increase of recruiting adhesion molecules and chemokines, although levels were always lower compared to the infarct zone. In a very recent study by Ye et al. [71] spatiotemporal recruitment of monocytes was imaged with combined (19)F/(1)H magnetic resonance, in vivo in a rat model of reperfused MI. Blood monocytes were labeled by intravenous injection of 19F-perfluorocarbon emulsion 1 day after MI. The distribution patterns of monocyte infiltration were correlated with the presence of microvascular obstruction (MVO) and intramyocardial hemorrhage assessed by MRI. Specifically, monocyte recruitment was highly reduced in MVO areas and that could be a plausible mechanism for delayed healing and worse functional outcomes. Therefore, monocyte recruitment in MI with MVO could be a potential diagnostic and therapeutic target that could be monitored noninvasively and longitudinally by 19F/1H MRI in vivo [71].
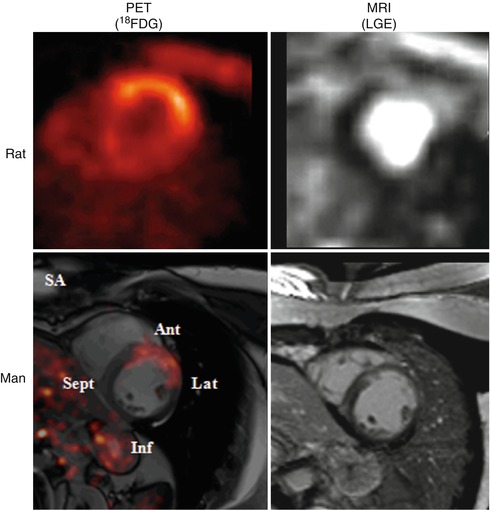
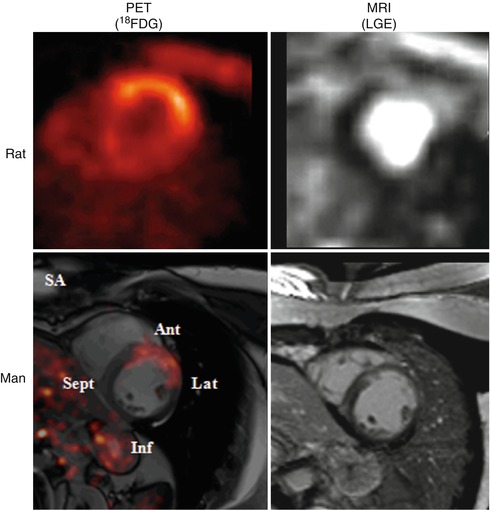
Fig. 23.7
FDG PET imaging in rat and man shortly after myocardial infarction and reperfusion corresponding to inflammation and/or a change in myocardial metabolism in the anterolateral region. The LGE MR images in the same area show a different volume of distribution for LGE pointing to local edema. For the rodent images, a preclinical PET and a clinical MRI was used. The human data were created on a fully integrated PET/MRI system. LGE late gadolinium enhancement, SA short axis, Ant anterior segment, Lat lateral segment, Inf inferior segment, Sept septum
At a preclinical level, quantitative radionuclide imaging of MMPs activity that is correlated with extracellular matrix degradation is now feasible using radiolabeled molecules targeting MMPs. This can be performed at different time points in combination with radionuclide (ECG-gated MPI SPECT) or non-radionuclide based techniques (MRI) for monitoring both scar size and LV volumes changes over time thus introducing a molecular/mechanical imaging approach that could help to assess in a longitudinal fashion the relationship between enhanced MMPs activation after MI and altered regional myocardial deformation. In the aforementioned study of Lee et al. [66] in mice, which employed 18F-FDG PET-MRI imaging for assessment of INFL, assessment of the activity of MMP-2 and MMP-9 was also performed using an activatable fluorescence reporter. It was found that MMP activity was significantly increased in non infarcted myocardium, suggesting that monocyte recruitment to the remote zone may contribute to post-MI dilation, although the precise cellular contribution of MMPs activity is unclear at this point.
Renin Angiotensin–Aldosterone System (RAAS) is another attractive imaging target due to its key role in various cardiac pathologies, including post-infarct LV REM. In a murine model of MI, investigators have used both a fluorescent angiotensin peptide analog and 99mTc-labeled losartan, an angiotensin II type 1 receptor (AT1R) blocker. Uptake of the angiotensin-targeted fluorescent compound was seen in the infarct area at 1–12 weeks after MI using an open chest imaging preparation, with maximum uptake within the heart seen at approximately 3 weeks after infarction. Subsequent histopathologic analyses suggested specificity for myofibroblasts within the infarct region in the weeks after the infarction. The investigators also performed in vivo micro-SPECT/CT with 99mTc-labeled losartan, demonstrating the feasibility for in vivo imaging of AT1R in the heart [72]. The potential of PET for AT1R imaging has also been investigated. The novel ligand 11C-KR31173 has been tested in a rat model of ischemia and reperfusion, where regional AT1R upregulation was detected in the infarct area. Subsequent testing of the same PET tracer (11C-KR31173) was performed in pigs 3–4 weeks after experimental MI [73] (Fig. 23.8). Ex vivo validation was carried out by immunohistochemistry and polymerase chain reaction. After MI, 11C-KR31173 retention corrected for regional perfusion, revealed AT1R up-regulation in the infarct area relative to remote myocardium, whereas retention was elevated in both regions when compared with myocardium of healthy controls. Postmortem analysis confirmed AT1R up-regulation in remote and infarct tissue. These early studies suggest that accurate quantification of AT1R overexpression by novel imaging techniques as mentioned above, if feasible, may become a helpful tool not only for identifying patients at risk for developing LV REM and HF after MI, but potentially for optimizing anti-RAS therapy.
< div class='tao-gold-member'>
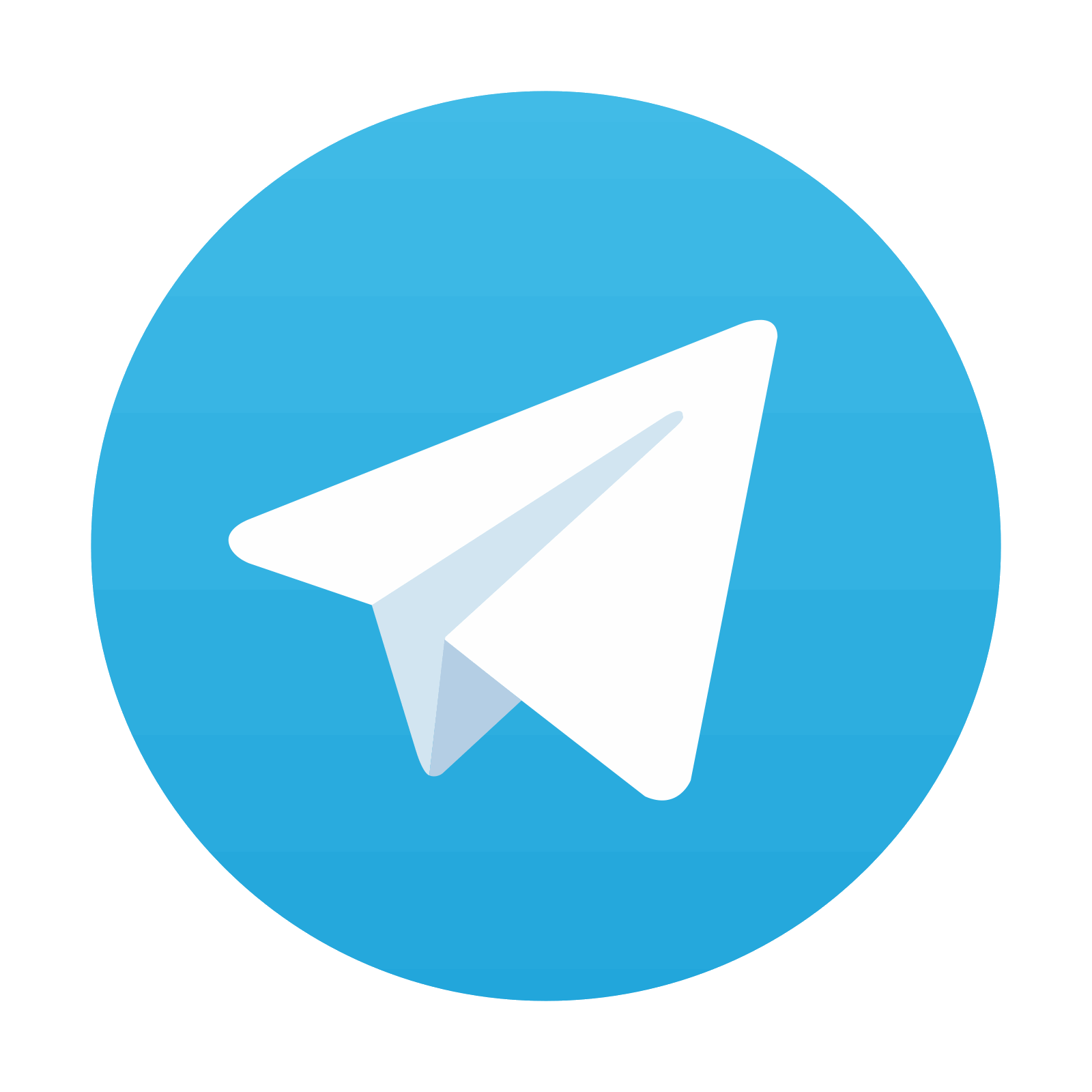
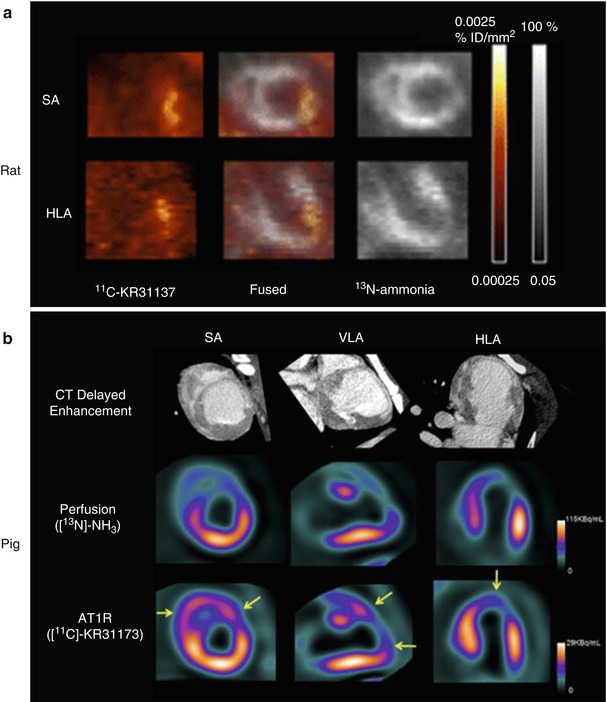
Only gold members can continue reading. Log In or Register a > to continue
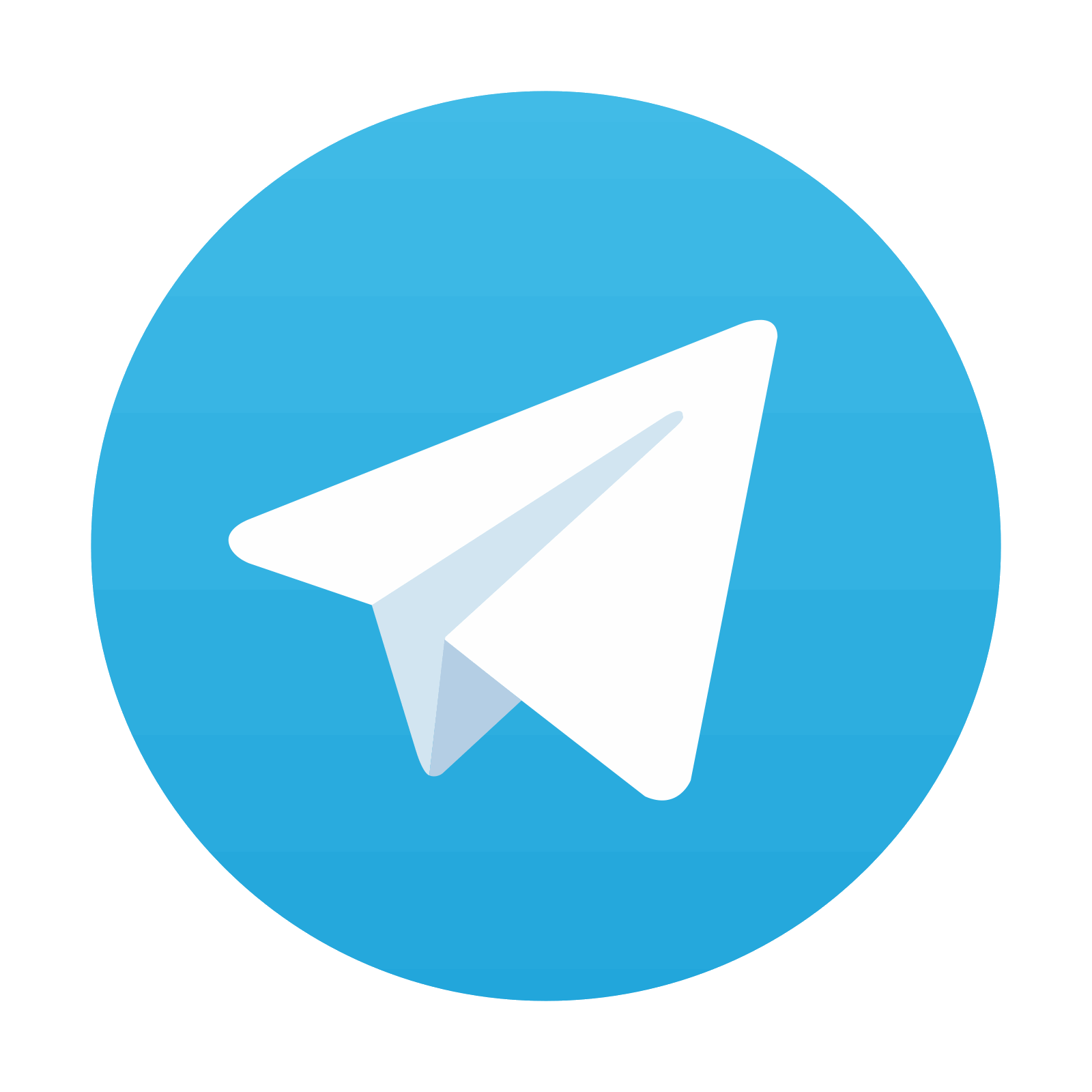
Stay updated, free articles. Join our Telegram channel
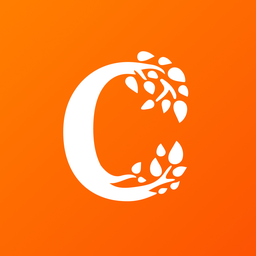
Full access? Get Clinical Tree
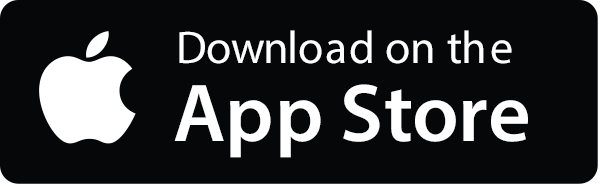
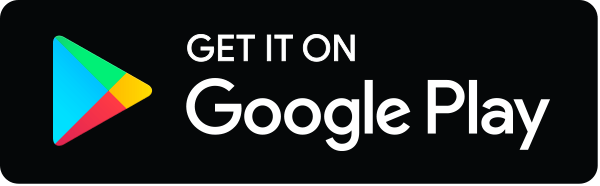