Chapter 11 Cardiovascular adaptations to chronic exercise
After reading this chapter, you should:
From the issues discussed in previous chapters it will be clear that one’s capacity to elevate cardiac output could be improved by increasing blood volume, by reducing resting heart rate (that is, increasing heart rate reserve) or by increasing stroke volume. It should also be remembered that cardiovascular capacity to service muscle metabolism is normally limited by the simultaneous thermoregulatory demands. Therefore, exercise capacity will be increased by minimizing hyperthermia and the associated encroachments on muscle blood flow and plasma volume that result from cutaneous vasodilation and sweating.
All of these adaptations occur, but they have very different timecourses. An initial increase in work capacity linked to increased cardiac reserve occurs over a matter of several weeks’ training, but the achievable cardiac output continues to rise over the succeeding months so that with any given training schedule, peak performance, as determined by cardiovascular efficiency, will not be reached until after around 9–12 months (Fig. 11.1).
BLOOD-VOLUME ADAPTATIONS
Red cell volume
The changes in plasma volume described above produce an initial fall in haematocrit, but with continued training there is, over the ensuing several weeks, increased erythropoiesis that restores the concentration of red cells to its previous level. The likely stimulus for increased red cell production is the recurrent renal medullary hypoxia associated with renal sympathetic activation, which results in release of erythropoietin from medullary tubule cells.
Collectively, the changes in blood volume constitute the major cause of the rise in cardiac reserve that is seen over the first 3–4 weeks of training (Fig. 11.1). As we shall see below, increased heart rate reserve also has a role during this period.
CARDIAC ADAPTATIONS
Heart rate reserve
Resting heart rate often begins to fall after the first few sessions of regular dynamic exercise and may be around 10 beats/min lower than the pretraining value after 2 weeks of training (Murray et al 2006). This confers an immediate advantage on exercise capacity, since it increases heart rate reserve. A 20 year old with a resting heart rate of 70 beats/min can theoretically increase cardiac output by (200–70/70) or 1.85-fold by tachycardia alone: with a resting heart rate of 60 beats/min this increase becomes (200–60/60) or 2.33-fold. Thus, in order to undertake any given submaximal workload, the trained individual needs only to increase heart rate (and, therefore, cardiac workload) by (1.85/2.33) or 80% of the amount needed before training.
In individuals who train intensively for prolonged periods, much greater degrees of bradycardia develop due to increased vagal tone, such that resting heart rate may be as low as 35 beats/min. This obviously confers a far larger cardiac reserve, with the 20-year-old athlete being theoretically able to increase his cardiac output by (200–35/35) or 4.7-fold using tachycardia alone, and reducing his cardiac workload for a given submaximal work intensity to (1.85/4.7) or 40% of that in an untrained age-matched individual. This more dramatic bradycardic effect of prolonged training is secondary to structural adaptation of the heart (see Cardiac hypertrophy, below).
During incremental dynamic exercise, it has been found that many trained athletes do not produce linear increases in heart rate up to their age-limited maximum. Instead, the slope of the heart rate/work curve flattens at around 85% (Lepretre et al 2005). Since this workload corresponds approximately to the anaerobic threshold, the heart rate deflection point has been adopted in a number of centres as an easy, non-invasive monitor for setting training workloads. It has also been suggested that the deflection infers some advantage on athletes by allowing greater utilization of their capacity to increase cardiac output by increasing stroke volume, although no firm evidence base for this exists. Regardless of whether or not the phenomenon has a value, the mechanisms that underlie it are unknown, and its usefulness as a training aid is limited by its variable occurrence even in trained athletes.
Cardiac hypertrophy
Like skeletal muscle, the myocardium responds to chronically increased workload by muscle cell growth, resulting in what is traditionally known as the ‘athlete’s heart’ (Iglesias Cubero et al 2000). As in skeletal muscle, the pattern of growth varies depending on whether the increased work is dynamic or resistive. Increased ventricular filling (increased preload), with no change in outflow resistance, results in moderately thickened muscle around an enlarged ventricular chamber (eccentric hypertrophy). By contrast, increased resistance to outflow (increased afterload) due, for instance, to increased peripheral resistance, leads to a markedly thickened ventricular wall with no alteration of chamber size (concentric hypertrophy) (Fig. 11.2).
Chronic dynamic exercise exerts both of these effects on the heart; the increased preload, because of increased venous return, and the increased afterload, because of the pressor response to central command. Not surprisingly, therefore, the initial structural adaptations to dynamic training involve elements of both types of hypertrophy. In the right heart, little or no afterload increase takes place because the pulmonary vasculature is exempted from sympathetic vasoconstrictor influences and, in fact, pulmonary vascular resistance falls during exercise due to better ventilation/perfusion matching (see Chapter 8, p. 95). The right ventricle, therefore, shows uncomplicated eccentric hypertrophy. On the left side, however, there are increases in both preload and afterload, which induces some degree of concentric hypertrophy and limits the expansion of left ventricular chamber size until after around 6–9 months training. Thus, the full benefits of cardiac adaptation, in terms of increased stroke volume, are not evident until after this period.
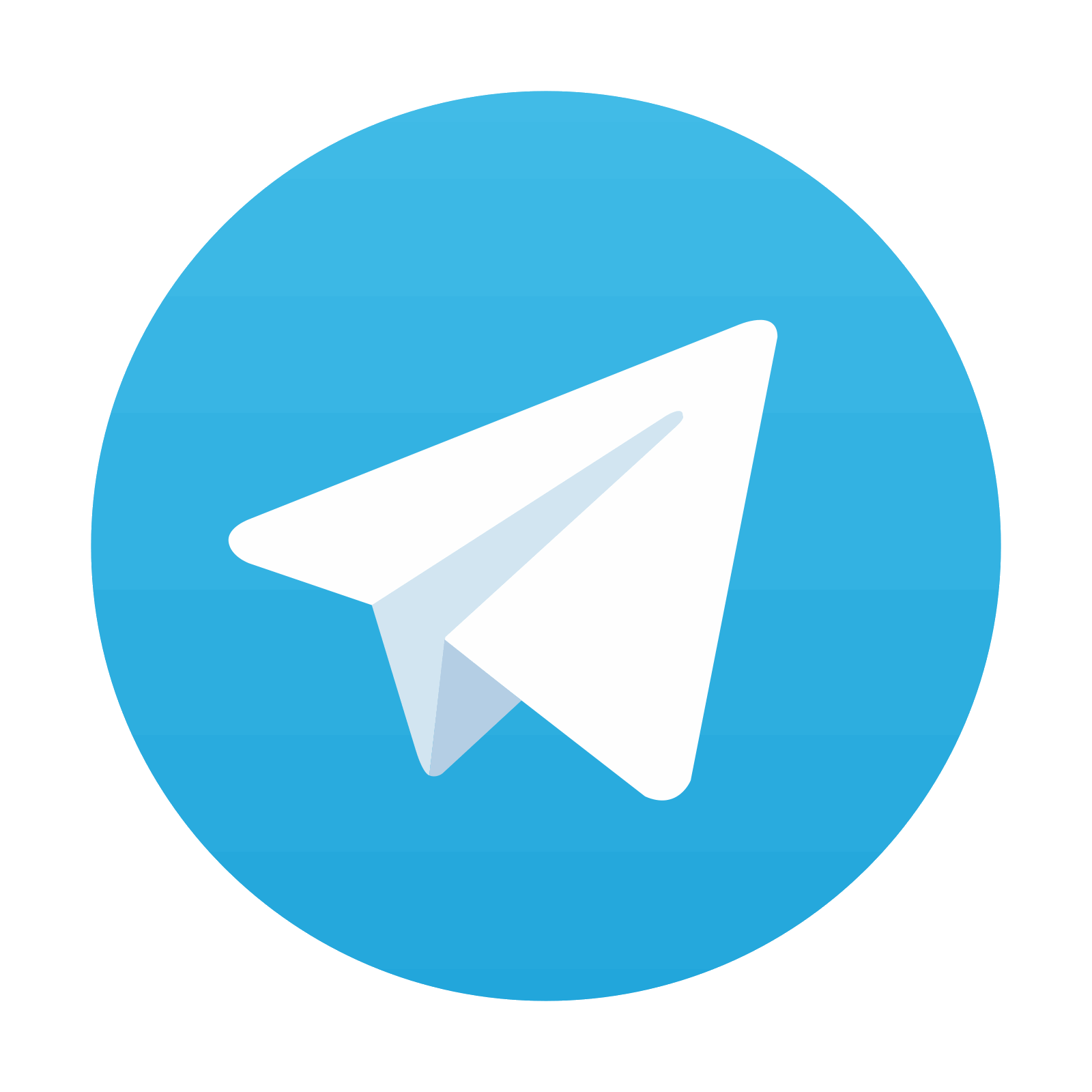
Stay updated, free articles. Join our Telegram channel
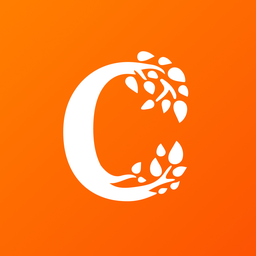
Full access? Get Clinical Tree
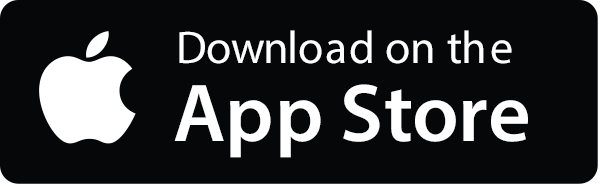
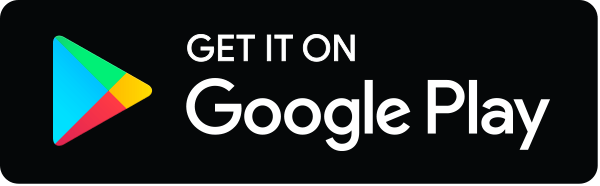