(1)
Kantonsspital Aarau, Medizinische Universitätsklinik, Aarau, Switzerland
Keywords
Diuretic resistanceCardio-renal cross-talkIntrarenal and intraglomerular hemodynamicsRenal perfusion/blood flowRenal hypoperfusionRenal autoregulation/impaired (altered) renal autoregulationMicrovascular dysregulationRenal venous pressureSystemic venous congestionRenal venous congestionNeurohormonal activationEndothelial dysfunctionRenal perfusion pressureDiureticsVasopressors, e.g. noradrenaline(Renal) autoregulatory threshold7.1 Definition
To express and to outline the special relationship observed between the heart and the kidneys in health and in malady, several characterizations and definitions have been proposed [1]. The knowledge of a specific interrelation between these two organs dates back as early as the seventeenth century BC, where in the Egyptian “Book of the Dead” one can find: “Homage to thee, O my heart! Homage to you, O my kidneys” [2]. In any case, traditional Chinese medicine already recognized and described a disorder termed “heart and kidney failing to link”, suggesting a close connection between kidney and heart dysfunction [3].
As such, the most recent and currently used definition is actually based on Ronco [4], who elaborated the most operational and practical determination of that interaction. The consensus conference of the Acute Dialysis Quality Initiative (ADQI), held in September 2008, compiled the following definition and characteristics of cardiorenal syndrome (CRS) [5]:
Cardiorenal syndrome, a complex disorder of both, the heart and the kidneys [6, 7], may be defined as “disorders of the heart and the kidneys whereby acute or chronic dysfunction in one organ may induce acute or chronic dysfunction of the other” [5].
7.2 Epidemiology and Prognostic Issues
Type 1 CRS is found to arise in up to 45% (incidence between 19 and 45%) of patients admitted with ADHF displaying acute kidney injury (AKI) [9–12]. The occurrence of AKI, attributed to AHFS, is indicative of an even worse prognosis, as it is associated with higher all-cause and cardiovascular mortality in both the short- and long-term perspective, and a prolonged hospitalization [10, 13–17]. Risk factors to develop AKI due to AHF include: a history of diabetes mellitus, severity of cardiac dysfunction on admission (the more severe heart failure the higher the risk to develop AKI) [14, 18], use of high dose diuretics (frusemide dose >100 mg/24 h, or use of high dose thiazides), vasodilator therapy or application of higher radiocontrast volume [9, 16, 19, 20]. Most cases of AKI occur and develop within 3–5 days after admission [9, 10, 21].
7.3 Clinical Issues and Diagnosis
Rapid worsening of renal function in the setting of AHF, resulting in volume overload, low CO and poor response to diuretic treatment (the latter known as “diuretic resistance”), are characteristic features of CRS, type 1 [4, 22]. Typically, signs and symptoms of fluid retention are present in the clinical picture with pulmonary (rales) and systemic congestion [9, 10, 23], while laboratory findings indicate elevated creatinine and nitrogen urea plasma concentrations [4]—see Chap. 2, BUN with prognostic validity [24].
Of note, patients with worsening renal function more often suffer from hypertension and complain about fatigue [9, 10, 23].
Following and applying the definition, the diagnosis of CRS type 1 requires incipient or worsening renal function attributed to, and induced by, primarily acute heart failure [7, 25]. Several tools to assess, to recognize and to classify renal dysfunction have been proposed and used in studies. I recommend to follow the definition of the RIFLE [26], respectively the AKIN classification [27], rather than to apply surrogate markers like the ratio of urine production to diuretic dose applied [28], or even newer biomarkers like NGAL or cystatin C (the latter arguably being a suitable indicator of high risk patients) [29–31]. The newer markers are not yet common daily practice, are not generally accepted by practitioners, and may need further evaluation in the context of cardio-renal syndrome [32, 33]. Applying AKIN allows for greater standardization of data, future (epidemiologic) studies and embeds CRS, type 1, into “the broader context of AKI” [34].
Impaired renal function, in the presence of AHF, is basically suggestive of altered renal perfusion [35], unless proven otherwise. Subsequently, either low cardiac output, and/or particularly the much more common increased renal venous pressure, has to be considered and the patient should be examined for this [36]. Renal venous congestion may distinguish acute CRS, type 1, from other etiologies of AKI [37]. Specifically, drug-induced renal functional alterations need to be taken into consideration in a differential diagnosis [38].
The poor response to diuretics (diuretics are the cornerstone and standard therapeutic approach in CRS type 1 [39, 40]) has diverse aetiologies [4, 41, 42], and the persistence of signs and symptoms of heart failure, despite suitable and increasing dosages of diuretics, is referred to as “diuretic resistance” [43].
Unfortunately, there is no generally accepted definition of diuretic resistance. The most commonly cited definition of diuretic resistance is: “a failure to decongest despite adequate and escalating doses of diuretics” [42]. The pathomechanisms involved and responsible for the poor effect of diuretic medication include: compromised renal blood flow (RBF) [43], altered enteral drug reabsorption [44, 45], reduced glomerular filtration (as loop diuretics act best from the luminal site) [46, 47], low albumin concentrations (impair uptake and secretion of active frusemide) [48, 49], and increased levels of urea nitrogen and other organic acids competitively hampering diuretic availability on the site of action [50, 51].
AKI related to AHF is most common in up to 60% of all patients with pre-existing renal dysfunction [11, 17], as is diuretic resistance in patients with pre-existing (chronically) impaired renal function [41, 52]. Predisposing issues to develop AKI due to AHF are: obesity [53, 54], diabetes [9, 55], hypertension [55–57], anemia [58, 59], and of course nephrotoxic drugs and even the medication applied to treat AHFS [4, 34, 38]. As such, particularly contrast agents, as given in case of coronary angiography, are a frequent precipitant of type 1 CRS [60–62].
7.4 Pathophysiology
The close interrelation and the interactions between the heart and the kidneys have been traditionally related to hemodynamic issues [63–65], as cross-talk between the two organs is physiologically necessary to regulate and to care for physiologic circulatory conditions and fluid and electrolyte homeostasis [1, 5, 65–68]. Cross-talk between organs is, in general, essential and indispensable to assure and maintain in vivo homeostasis, physiological and smooth functioning of the organism [5, 69]. The communication between the heart and the kidneys is of bidirectional nature, using several pathways available to notify, give feedback and impact on each other [43, 66, 69]. In the setting of malady, the injured, dysfunctional organ, may affect the other via various complex humoral, metabolic, and cell-mediated pathways [69]. As such, acute heart failure has a direct impact on kidney function (and vice versa in case of acute renal dysfunction) [4, 5, 7], by immediate precipitation of disrupted and toxic cell signaling promoting distant organ malfunction and/or structural alterations [70].
In detail: immune and somatic cell signaling may be substantially altered; the inflammatory cascades including augmented cytokine release and features associated with endothelial dysfunction are activated; enhanced neurohormonal (sympathetic and renin-angiotensin-aldosterone) drive and modified heart- renal reflexes (e.g. Henry-Gauer reflex) are described; neutrophil migration, leukocyte trafficking, enhanced oxidative stress and disturbed redox homeostasis are verified; non-osmotic release of arginine vasopressin (disturbed hypothalamic-pituitary axis), and cell apoptosis are all potentially able to markedly affect distant organ function and structure, particularly the renal tubular epithelium and the renal vascular endothelium [1, 8, 22, 36, 66, 71–73].
As a main result, intrarenal and intraglomerular hemodynamics are substantially altered, affecting GFR [4, 5, 8, 36, 74–76], and fluid and electrolyte homeostasis [8, 71, 77, 78], and thus renal dysfunction, AKI, may apply. Accordingly, it is not astonishing, that traditionally cross-talk between heart and kidneys has been exclusively considered to be a hemodynamic feature [63–65, 79]. The attenuated GFR, and the fluid and electrolyte derangements are a consequence of altered renal hemodynamics, attributed to acute or chronic heart failure [8, 77, 80, 81], as Guyton explained: “Combined heart and renal failure in terms of interactions between cardiac filling and contractility, renal function, blood pressure and blood and extracellular fluid volumes” [67]. Indeed, altered renal function can, by all means, be related to changes in renal blood flow, and renal and glomerular perfusion pressures arising from incipient AHF [82]. As such, renal dysfunction related to AHFS has been attributed to renal hypoperfusion following low cardiac output [4, 22, 66, 74, 75, 83].
Renal blood flow, and subsequently the glomerular filtration rate (the latter being a hallmark of renal function which is decisively depended on renal perfusion/perfusion pressure and RBF respectively [84, 85]), are regulated by a very complex interplay between renal and systemic vascular resistance, CO and effective circulating blood volume, and intrarenal and intraglomerular hemodynamics. Thus, conditions determined, modulated and affected by miscellaneous factors and features including: renal autoregulation (with its two components, tubuloglomerular feedback and myogenic response of renal vasculature [84, 86, 87]), circulating and local hormones, paracrine factors, as well as endothelial and renal neurohormonal (sympathetic and renin-angiotensin-aldosterone system, baroreceptor-mediated neuronal) effects and replies [86, 88–94]. Impaired renal perfusion activates at least some (depending on the severity of hypoperfusion) of these mechanisms to compensate for hypoperfusion, largely facilitating renal and systemic vasoconstriction, and sodium and water retention in order to restore renal and systemic (as there will be, in turn, macrocirculatory effects [88, 95]) perfusion pressure, systemic perfusion in general and as such renal blood flow [4, 7, 74, 83, 96]. However, in case of mild to moderate reductions in CO and/or intravascular volume (the effective circulating blood volume), basically renal autoregulatory mechanisms (autoregulation is considered being primarily a pressure-mediated mechanism [97]), will promote a gradual vasodilation (mediated by myogenic response and vasodilating agents, such as prostagalandin I2 and NO) of the afferent, preglomerular arterioles, atoning for the diminished blood flow offered to the kidneys, by adapting the renal perfusion to the altered conditions and thus maintaining RBF [88, 90, 98–100]. Tubuloglomerular feedback (TGF) fine-tunes renal perfusion and GFR by co-adjusting the tone of afferent arterioles (vasodilation) via local mediators such as NO or adenosine [86, 89], and of vas efferens (mild vasoconstriction in order to maintain or restore glomerular filtration pressure), largely mediated via local renin release (of the macula densa due to diminished chloride concentration there), and thus concomitantly, locally generated angiotensin II [89, 93, 101], thereby contributing to restore and/or to preserve GFR. Additionally, salt and water retention ensues [102, 103] substantiating the adjusting measures. Myogenic response and TGF may be modulated by neurohormonal effects via paracrine and endocrine paths, as well as neurally-mediated reflexes and responses [86, 93, 104–108].
The circumstances are clearly different in case of severe AHF with markedly reduced CO, often accompanied by significant hypotension, and/or if compensatory mechanisms cannot resolve the compromised renal blood flow: Markedly reduced CO and thus effective circulating blood volume, or otherwise diluted effective circulating fluid volume, diminishes renal blood flow and will subsequently reduce renal perfusion pressure [98, 107]. This affects and substantially blunts, or disrupts, autoregulation, as soon as renal perfusion pressure drops below the autoregulatory threshold [98, 109]. Renal autoregulation is acknowledged to be attenuated, disturbed or even disrupted in heart failure, due to hemodynamic changes, but also related to endothelial dysfunction (see below) [8, 22, 37, 110–112]. As a response, a marked activation of the neurohormonal systems arises with a perceptible effect of an elevated sympathetic drive, and a strongly stimulated RAAS with substantially increased levels of angiotensin II (A II) [113, 114]. This is the result of attenuated and reduced stretch of the baroreceptors of the renal vasculature (e.g. vas afferens), due to an attenuated renal blood flow, consecutively considerably stimulating the neurohormonal systems [6, 43, 65, 80]. Its response (neurally/reflectory-mediated release of mediators like A II, NA, endothelin-1, vasopressin-arginine, etc.) promotes combined constriction of vas afferens and vas efferens, increasing their tone and total intra-renal vascular resistance [71, 93, 107]. Increases in the tone, and thus resistance, of vas afferens, and (general) increases in renal vascular resistance, are associated with reduced renal blood flow and glomerular filtration pressure, and subsequently a fall in GFR [93, 115–118]. Furthermore, changes in the tone of vas afferens and efferent are discordantly in those circumstances, as the afferent arterioles constrict relatively stronger than the efferent ones, since vas afferens had been initially dilated and is also more densely innervated by sympathetic nerves (three times more) compared to vas efferens [119]. However, as the effective filtration pressure is mainly affected by the ratio between the tones of afferent and efferent arteriole [76, 88, 90, 120], this ratio shortens as the tones of both arterioles approach each other. In consequence, a generalized vasoconstrictive environment within the renal vascular bed is engendered [88, 118, 121], and renal function evidently affected. Furthermore, subsequently a considerable volume expansion, namely due to the effects of the sympathetic nervous system, aldosterone and arginine vasopressin, applies [80, 108, 122–125], which may, in turn, restore renal perfusion [102, 103]. However, this volume expansion is basically achieved at the expense of a substantial fluid overload, and in any case, the enhanced renal water, and particularly sodium reabsorption, provokes extracellular fluid expansion, as well as systemic and pulmonary congestion [71, 78, 80, 122]. Therefore, these hemodynamic alterations cause a bidirectional coupling, as renal failure due to AHF causes fluid retention which aggravates heart failure, and thus may provoke further reductions in arterial blood pressures thereby worsening renal perfusion even more [1].
Moreover, if GFR cannot be restored by the applied compensatory mechanisms, the kidneys are at high risk of ischemia and ischemic insults [107]: As with reduced glomerular capillary pressure, the post-glomerular vessel network may be under-perfused, thus tubular ischemia potentially applies and if evoking structural tubular injuries, acute tubular necrosis (ATN) may arise [126]. Furthermore, local differences in the intensity of the increase in renal vascular resistance are observed, resulting in diverse regional perfusion within different kidney areas [85, 95, 127, 128]. Endothelial dysfunction arises, and with limited NO bioavailability, endothelial-dependent vasorelaxation is mitigated [128], thus, microcirculatory failure applies. As such, substantially altered intra-renal microcirculation ensues [95, 129, 130], creating disproportionally modified, diverse local intra-renal blood flows [100, 131–134], promoting hypoxia/ischemia in predisposed areas, particularly the outer medulla [95, 132, 135, 136]. Subsequent reperfusion injuries may ensue [90, 132, 137].
Autoregulation, in general, refers to the ability of a vascular bed to adjust its tone to maintain a constant blood flow during changes in perfusion following variations in arterial perfusion pressure [138, 139]. “Autoregulation is largely and essentially a local mechanism of control of blood flow” [140]. Thus, autoregulation, as a crucial component determining microcirculatory hemodynamics [110, 141–143], provides a rather constant blood flow, and particularly, an appropriate blood flow distribution over a wide range of different perfusion pressures, ensuring that oxygen and nutrient supplies meet actual metabolic demand of each organ, region and tissue area [144, 145]. A well performing autoregulation is obviously a critical element in a proper renal perfusion arrangement, as altered and affected renal autoregulation applying in the context of AHFS has a substantial impact on renal function [8, 76, 97]. Even in case of adequate CO, as found in the vast majority of AHF patients, GFR reductions are demonstrated in quite a number of patients, arguably attributed to impaired renal autoregulation [76]. Indeed, renal autoregulation is basically mediated by changes in the tone of vas afferent [97]. As such, uneven renal blood flows are considered to be due to attenuated or impaired autoregulation [76], and CRS type 1 is suggested to at least partly develop secondary to autoregulatory dysfunction [76].
Lowering elevated BPs in acutely decompensated heart failure patients may affect autoregulation and renal function, although BPs are therapeutically “only” reduced to normal ranges [146, 147]. Drugs affecting renal autoregulation may contribute to blunted autoregulatory effects, including loop diuretics, renin blocking agents or non-steroidal anti-inflammatory agents [148]. Hence, GFR remains stable, unless renal autoregulation is attenuated or impaired, like in case of severe hypotension and/or markedly reduced CO, or in case renal autoregulation is afflicted, as in the setting of AHFS [8, 76, 97, 98]. Accordingly, only as long as renal autoregulatory capacity is uninterrupted, GFR will be maintained, despite reduced renal perfusion, resulting from mild to moderately impaired CO, reduced effective circulating volume or otherwise diminished intravascular filling [97, 98, 146, 147].
Renal autoregulation is closely related to, and dependent on, endothelial cell function: Endothelial cells are acknowledged to play a central role in the regulation of the microcirculation [149–151]. They exert relevant influence on vasomotor tone [143, 152] (via a dedicated collaboration and cross-talk with the vascular smooth muscle cells [150, 153, 154]), show cross-talk among themselves (communicating upstream information about the hemodynamic situation and constitution in the downstream areas (backward communication)), and as such, modulate and adopt local blood flows [110, 155, 156]. Accordingly, they decisively contribute to and arrange for a well-functioning microcirculation [149–151]. In low flow conditions, pro-inflammatory and pro-thrombotic properties are expressed [157]. It is crucial for flow adaptions that endothelial cells align with actual conditions and any disordered alignment, as may be present in case of disturbed blood flows, leaves the inflammatory pathways activated [150]. A compromised endothelial function is known to impair local vascular autoregulation and to provoke perfusion mismatch [158–161]. Unfortunately, endothelial cell function is reported to be afflicted in AHFS [112, 162–164]. Correspondingly, a well-performing autoregulation is closely related to, and also markedly dependent on, endothelial cell function, because the endothelium plays an obligatory role in cardiovascular homeostasis by regulating vascular tone (and cardiac function as influencing ventricular load by vascular stiffness [162, 165] and coronary and myocardial perfusion and thus ventricular function [166]), adjusting vascular permeability, preserving blood fluidity [167], and is particularly central to functions of the microcirculation [149].
However, by far the vast majority of patients admitted due to AHF are adequately perfused, with an at least reasonable CO and fair blood pressures caring for preserved renal blood flow and autoregulatory capacity—in fact, far less than 10% of all AHFS exert compromised organ perfusion [168–175]. As such, this traditional view of renal hypoperfusion being mainly responsible for incipient renal dysfunction in AHFS has been warrantable challenged [37, 176, 177]. Indeed, no correlation has been found between baseline renal function and CO/CI [19], an improvement in cardiac index does not translate into improvement in renal function [174, 178], and even patients with relatively normal systolic function (those with preserved ejection fraction and/or preserved CO) are often presenting with, or develop, impaired renal function [171, 179, 180]. Moreover, worsening renal function can be found in a similar range in patients with preserved and those with reduced systolic function [181], and most AHFS patients are admitted with elevated BPs rather than being hypotensive [41]. Recent trial results present convincing evidence, that in those patients predominantly venous congestion is the main reason for (and cause of) renal dysfunction [169, 174].
That elevated renal venous pressures may affect kidney function has already been described more than 75 years ago [79, 182]: In a dog model, Winton [79] recognized a deterioration of urine generation with renal venous pressures above 20 mmHg and even a suspended urine formation at pressures ≥25 mmHg. Furthermore, he expressed a relationship between elevated central venous pressures and reduced renal blood flow, indicating that renal blood flow decreases with the decline in pressure gradient between vas afferent and vas efferent, probably induced by an increase in vas efferent tone [79]. Later on, extrinsic compression of abdominal veins due to intra-abdominal hypertension were also reported to compromise renal function [183, 184], which has in the meantime be confirmed by several studies [185, 186]. Firth showed a direct transmission of elevated central venous pressures to the renal veins, attenuating GFR, as increased renal venous pressure was accompanied by a drop in glomerular perfusion pressure—a dysfunction that may recover, if enhanced pressures are resolved [187]. Gottschalk and Mylle [188] demonstrated that in case where renal venous pressure exceeds 15 mmHg, a linear increase in peritubular capillary and intratubular pressures arises. However, every increase in intratubular pressure directly diminishes net ultra-filtration pressure, as enhancing the pressure within the Bowman’s space, which opposes glomerular filtration pressure, and subsequently attenuates GFR [1, 76, 97, 189]. Raised systemic and renal venous pressures, with concomitant congestion of the renal venous system, are thought to cause extravasation and congestion of the kidney [82, 177]. Since the kidney is surrounded by a tight non-distensible capsule [82, 177], subsequent interstitial intra-renal pressure increases in case of elevated renal venous pressures [190–193].
Consecutively, renal parenchymal hypoxia, tubular dysfunction, due to tubular obstruction, or even collapse concomitantly opposing glomerular filtration pressure [97], and activating the RAAS may apply, promoting a decrease in GFR [97, 191–193]: Elevated venous pressures, in any case, reduce the trans-renal perfusion pressure (a decrease in arterio-venous pressure gradient occurs with increasing venous pressures within the renal vessel system), will provoke a diminished renal blood flow [22, 97], and may distend the venule network surrounding the tubules of the distal nephron, causing tubular compression, obstruction or even collapse of the tubules (at least as long as the pressure of the ultrafiltrate does not exceed venular pressure [79]) ensue [107, 177]. Subsequently, net glomerular filtration pressure is lowered, and backleak of the ultrafiltrate into the interstitium may occur, the latter potentially leading to an increase in the interstitial pressure [107, 177].
With increasing renal venous pressure, neurohormonal activation ensues. As such, increasing renal and systemic angiotensin II concentrations are demonstrated to accompany increasing renal venous pressures [190, 194], leading to (further) decreases in GFR, enhanced proximal tubular sodium and water reabsorption (aggravating heart failure and renal venous and intra-renal interstitial pressure elevation and congestion), and stimulated sympathetic drive [71, 125, 192, 195]. Angiotensin II and sympathetic activity affect arteriolar tone and thus, impact on perfusion and afferent and efferent glomerular pressures [125, 190].
This concept is further considerably supported, and profoundly substantiated, by results demonstrating an association between increased central venous and right atrial pressures, attributed to acute decompensating or chronic heart failure, and worsening renal function. Moreover increasing central venous pressures go along with an increased mortality rate in that patient group [174, 196, 197]. Beyond this, elevated central venous pressure is reported to be associated with higher baseline createnine serum concentrations [197] and tricuspid regurgitation, attributed to heart failure, and shows a relationship with renal dysfunction [198]. Damman finally verified that in heart failure patients, venous pressure is an independent determinant of glomerular filtration [169].
It is not definitely known how autoregulation responds to the increased renal venous pressure, however, renal autoregulation is considered to be affected by the above described hemodynamic alterations (renal hypoperfusion and renal venous hypertension), impairing autoregulatory effects and efficacy, or even provoking complete breakdown of autoregulation [8, 22, 76, 97]. It seems, and it is suggested that, due to the increased renal venous and interstitial pressures, not only the RBF will be attenuated, but that the myogenic response is strongly affected (while TGF is not relevantly impacted) and thus autoregulation impaired [199, 200]. Meanwhile, “systemic venous congestion” (and thereby renal venous congestion) is acknowledged to be “the major driver of acute cardiorenal syndrome (CRS, type 1), especially in severely elevated central venous pressure from RV dysfunction and/or tricuspid regurgitation” (associated with (acute) heart failure) [35, 76, 169, 174].
Haase [76] summarized the hemodynamic alterations potentially displayed, and to be anticipated in, CRS type 1 patients with respect and related to the clinical-hemodynamic profile assessed by physical examination at bedside as proposed by Stevenson [201] (and later resumed and established by Nohria and co-workers [202, 203]). This is currently the widely used and even endorsed (by AHA/ACCP and ESC) approach [204] to evaluate the predominant clinical-hemodynamic condition of AHF patients and seminal for the initial therapeutic approach and prognosis [202, 204–206]: Source [76].
Warm and dry | Warm and wet |
Discordantly ↓ RBF | Discordantly ↓ RBF |
Intra-renal microvascular dysregulation | Impaired intra-renal autoregulation |
↑ renal venous pressure | |
Cold and dry | Cold and wet |
↓ RBF | Discordantly ↓ RBF |
Impaired intra-renal autoregulation | Impaired intra-renal autoregulation |
↑ renal venous pressure |
Beyond the described hemodynamic issues, several non-hemodynamic features, namely the neurohormonal activities and the inflammatory and endothelial effects, are considered to be relevant contributors to, mediators of and communicators in the development of CRS, type 1, linking heart and kidneys and impressively demonstrating, how cross-talk and interactions work, and conditions/information are mediated [1, 36, 69, 83, 207].
As such, the renin-angiotensin-system is a typical example of the bidirectional impact, which the heart and the kidneys exert on each other, as well as being a connector of both organs [1, 35, 115]. Increased renal venous pressures [208, 209], diminished renal artery pressure [210], diluted sodium concentration in the distal nephron [211], and enhanced sympathetic discharge [210], all are demonstrated to be associated with, and are conditions of, the pathophysiology of acute and chronic heart failure and kidney afflictions [36, 78, 83, 212]. They have been shown to promote substantial renin release and thus activate the renin-angiotensin cascade [115]. Elevated renin secretion is characteristic of early biventricular heart failure, leading and contributing (via angiotensin II (A II)) to myocardial and renal dysfunction, and promoting edema formation [212]. Activation of the renin-angiotensin system allows to maintain glomerular perfusion pressure and glomerular filtration rate, despite reductions in cardiac output and/or low BPs, through preferential constriction of the efferent glomerular arterioles in patients with HF [213]. The biologically most active representative of the renin-angiotensin-system, angiotensin II, stimulates pro-inflammatory cells, thus induces the generation of reactive oxygen species (via the NADPH/NADH oxidase pathway [214, 215]) and pro-inflammatory mediators [216], and is, as such, coupled to the inflammatory path connecting both organs [1, 176]. A II causes and amplifies renal and systemic vasoconstriction, subsequently enhances LV afterload, diminishes renal perfusion, increases venous pressure and facilitates edema formation [22, 36]. Furthermore, A II (and the RAS) has been shown to be tightly linked to the sympathetic nervous system [217], whereupon signals of the sympathetic nervous system to the kidney are closely related to incipient CRS [218]. Not at least, A II causes aldosterone excretion, and hence promotes tubular water and sodium reabsorption [36]. Both, heart failure and renal failure are substantially influenced by (but also simultaneously facilitate) incitement of the inflammatory and oxidative path, adversely affecting both organs [176, 207]. Meanwhile, oxidative injury is recognized as a “common link between cardiac and renal dysfunction” [207] and the final common pathway in CRSs [36].
As already described, the vascular and cardiac endothelium is another feature, mediator, coordinator and conductor orchestrating inflammatory and vascular reactions and replies. It is not only A II which causes endothelial dysfunction (ED) [216, 220], but rather heart failure and chronic kidney disease are both independently associated with ED [221, 222]. Disrupted NO pathways and reduced NO bioavailability, affiliated with ED, are major issues in heart failure pathophysiology, substantially influencing renal function [207, 223–225]. ED may considerably affect renal autoregulation [158–161], as ED is also associated with oxidative stress (with effects on renal sodium management, systemic and renal hemodynamics [226–228], and glomerular glycocalyx barrier function [229, 230]) and the inflammatory cascade [1, 36, 176, 231], showing definite cross-links between both organs [72, 232–234]. Indeed, CRS may be considered as a low-grade inflammatory disease, attributed to an imbalance between immune system cell signaling [36, 235–237], and interleukin-6 (IL-6) has been identified as a complex cardiorenal connector [1, 238, 239].
Beyond the activated RAAS, ED, inflammation and ROS, the important role and interconnection of the sympathetic activation in the pathogenesis, pathophysiology and progression of heart (and renal) failure has already been stressed [240]. Enhanced sympathetic drive, by increasing afferent arteriole tone, mitigates RBF and GFR, and thus affects renal function [241, 242]. Other factors discussed as possible contributors include: gut ischemia and (consecutive) endotoxemia [243–245]; superimposed infections [246, 247]; iatrogenic effects (especially drug applied with kidney compromising effects) [248–251]; and a failure of counter-regulatory mechanisms (e.g. natriuretic peptides) dampening the depicted (compensatory) mechanisms and features [36].
To conclude, the pathobiology of the cardiorenal syndromes is complex and multiple mechanisms may be involved [4, 5]. The impact and the importance of each feature contributing may vary from patient to patient [7]. The pathophysiology of CRS, type 1, largely includes hemodynamic features such as diminished RBF and deficient renal perfusion pressure, increased intra-renal vascular resistance, as well as enhanced renal venous pressure (with concomitant renal venous congestion) [97], the latter being identified as the “major driver of acute cardiorenal syndrome” type 1 [36, 76, 169, 174, 252]. Altered renal perfusion in the setting of acute (and chronic) heart failure is attributed to and may be the result of impaired CO, combined with pre-glomerular vasoconstriction and renal venous congestion [253]. However, GFR (and thus renal function) remains stable unless renal autoregulation is attenuated or impaired, as may be (I) in case of severe hypotension and/or markedly reduced CO resulting in hypoperfusion, (II) when renal perfusion pressures are beyond the autoregulatory threshold, (III) and/or in case renal autoregulation is afflicted by features such as renal venous congestion, ED, diminished intrarenal perfusion, and altered (intra)glomerular hemodynamics, all apply in the setting of AHFS [8, 76, 97, 98]. As such, a proper working autoregulation is critical in renal physiology.
All hemodynamic factors are strongly related to volume retention and activated neurohormonal systems (sympathetic and RAAS) [122, 254]. Indeed, features associated with and contributing to CRS type 1 are sympathetic-mediated fluid redistribution, venous congestion, inflammation, and endothelial dysfunction [174, 255–257]. Venous congestion, enhanced neurohormonal activity, ED and inflammation are the main trigger, contributors, and mediators precipitating baseline renal dysfunction by altering intra-renal and intra-glomerular hemodynamics and by affecting renal auto-regulation [4, 36, 76, 252]. Moreover, type 1 CRS may, in fact, be also perceived as an inflammatory disorder, as the inflammatory pathway and associated features, namely ED and oxidative stress, markedly contribute to the pathogenesis, and inflammation is fundamental for the occurrence of distant organ damage [7].
7.5 Management
The management of CRS type 1 predominantly relies upon the approach by which acute heart failure is tackled [5, 32, 36, 43, 175, 207]. Specific renal requirements and issues need to be considered, before taking actions aimed at disrupting cardiorenal connections and dependencies, by applying multi-modal paths addressing the various underlying patho-physiologies [7, 107]. Restauration of physiological renal hemodynamics can be achieved in part by relieving the patient from congestion and symptoms, and further, any measures jeopardizing renal function need to be absolutely avoided [43, 76, 175].
Before it can be beneficial, it is strictly necessary, that any therapeutic measure used to approach AHF does not exert negative effects on kidney performance [258]. Accordingly, particularly nephrotoxic drugs like radiocontrast media, non-steroidal anti-inflammatory agents, and opiates altering renal hemodynamics (and thus impairing autoregulation and thereby negatively affecting kidney function), should be held off [60, 61]. Furthermore, adequate BP (MAP of ≥70–80 mmHg, with 80 mmHg being the target one should definitely aim for in patients with chronic hypertension [259, 260]) guarantees operating renal autoregulation and thus maintains glomerular perfusion [97]. Hypotension and/or intravascular underfilling have to be avoided, eliminated and prevented [147, 261].
7.5.1 Diuretics
The application of diuretics is the cornerstone in the treatment of AHFS [36] and CRS [22, 32, 36], but it is somewhat of a double-edged sword. They are important to resolving congestion and thereby improving patients’ symptoms and comfort in general, and with respect to CRS type 1 in particular, they address renal venous congestion and fluid overload, but unfortunately, they may unfavorably affect kidney function and further activate the neurohormonal systems [262–264]. As such, by reducing elevated central and renal venous pressures, the latter being a major driver of worsening renal function in AHF patients [36, 76, 169, 174], diuretics are an essential and effective feature in the treatment armamentarium [5, 22, 32, 36, 43]. Furthermore, Atherton [265] impressively demonstrated that in decompensated severe heart failure, with considerably elevated LVEDP, diuretics are not only very effective to relieve the patients` symptoms and to improve clinical and hemodynamic conditions, but are generally well tolerated, and do not worsen circulatory issues (primarily BP). Roughly 50% of all patients admitted to hospital suffer from biventricular failure, and thus the LV is relevantly compromised by pericardial constraint and ventricular interactions (specifically diastolic ventricular interdependence, DVI). Especially unloading of the right heart (thereby attenuating systemic congestion), will optimize LV filling and intraventricular pressure terms, subsequently facilitating LV performance and hence supporting macro-hemodynamics. In any case, no BP drop could be demonstrated, not even in patients without relevant pericardial constraint and DVI, thus no significant hemodynamic setback has to be anticipated if diuretics are applied in those patients.
However, in case of (intermittent) arterial underfilling—due to “overshooting” diuresis, following application of diuretics—renal perfusion may worsen, while the neurohormonal systems will be further activated [115, 262]. As such, the rate of fluid removal should not exceed the rate of fluid mobilization, and tissue fluid reabsorption rate is estimated to range between 12 and 15 mL/min [266, 267].
Early use of diuretics is reported to reduce mortality in severe AHFS, while systemic congestion (indicated by elevated central venous pressure) is related to worsened mortality in AHFS [196]. Effective and substantial decongestion is a decisive prognostic feature, and influences the evolution of the disorder. Incomplete decongestion rather than increasing createnine serum concentrations are associated with disease progression and worsens the chance of survival [268, 269]. On the other hand, a relationship between increased requirement of loop diuretics and increasing mortality has been demonstrated [250, 270, 271]. Thorough monitoring of diuretic use and effect is necessary [36].
Diuretic resistance, a specific issue [75], may complicate CRS [34, 43]. The underlying pathomechanisms are diverse [41, 42] and may include: compromised renal blood flow (e.g. hypotension and/or hypoperfusion) [43, 272], blunted intestinal absorption of the diuretic agent [44, 45], reduced glomerular filtration as loop diuretics act best from the luminal site [46, 47], low albumin concentrations (impair uptake and secretion of active frusemide) [48, 49], and increased levels of urea nitrogen and other organic acids competitively hampering diuretic availability on site [50, 51]. Accordingly, all features leading to reduced availability of the diuretic drug at the site of action (which is the thick ascending limb of the loop of Henle for loop diuretics, and the distal convoluted tubules for thiazide diuretics and metolazone, which is a thiazide-like drug, the latter commonly and preferably applied in CRS), have to be considered and should be addressed if possible [36, 41, 43, 273, 274].
The recommended dosage of diuretic medication at which it becomes effective varies widely [22]. However, either increasing the dosage of loop diuretics or adding a second-site diuretic agent [275, 276] (e.g. metolazone 10–20 mg bd/tds or hydrochlorthiazide 50–100 mg per day (in severe cases 100–200 mg per day [273])), is generally advised in case of diuretic resistance [22, 32, 82, 273]. As only 50%, or less, of frusemide is absorbed in case of systemic venous congestion and edema [277], i.v. application may overcome intestinal reabsorption difficulties [43].
Furthermore, since no significant differences in renal function have been observed when applying loop diuretics as several bolus injections or via continuous infusion, the kind of intravenous application does not matter [250].
Frusemide: 40–80 mg i.v., 80–160 mg may be required several times a day, e.g. tds or qds in case of moderate renal insufficiency, if renal impairment is severe, 160–200 mg, e.g. tds or qds. The maximal natriuretic response is reported to be achieved with i.v. bolus injections of 160–200 mg frusemide (or equivalent torasemide/bumtanide dosages) [278, 279].
Torasemide: 20 mg i.v., 20–50 mg tds in case of moderate renal impairment, 50–100 mg tds if severe renal insufficiency.
Bumetanide: 1–2 mg i.v., 4–8 mg in case of moderate renal insufficiency, 8–10 mg if renal dysfunction is severe.
Felker [250] examined the effect of different frusemide dosages, by applying to one group intravenously (either by continuous infusion or i.v. as a bolus every 12 h) the same dose of frusemide which these patients had previously, before admission, taken orally, while he gave the other group of patients, the high dosage group, 2.5 times the amount of oral dose. No significant differences were found between either groups and thus between the dosages, nor between continuous or bolus intravenous application, observed over a period of 72 h. However, although not significantly, the high dose group showed beneficial effects in secondary outcome criteria such as: relief of dyspnea and congestion, amount of weight loss, reduction of elevated cardiac biomarkers (natriuretic peptides), and a trend of a lower rate of hospitalizations, but also developed some mild degree of renal dysfunction which reversed within 1 week.
The addition of mineralocorticoid diuretics (MRAs) in an acute setting has not been examined. However, they are recommended in the guidelines for chronic heart failure therapy in low dosages [280, 281]—class I A recommendation [204]. They may be added, even to a combination of loop diuretics and thiazides [282, 283], in acute decompensations at “higher” dosages (50–75 mg daily—12.5 and 25 mg there is no natriuretic effect at all [284]), as smaller observational studies suggest, since MRAs may improve diuresis (in diuretic resistance) and thus the clinical condition of the patient [285, 286].
Note: Dose titration should in general be subject to effectiveness and/or the side-effects experienced [287].
Two randomized controlled studies (UNLOAD and RAPID-CHF) comparing ultrafiltration with diuretic medications, revealed a greater fluid removal and significantly fewer re-hospitalizations and unscheduled visits for heart failure in the ultrafiltration groups [288, 289]. However, weight loss within 24 h [289] and dyspnea scores [288] did not differ.
The result of a recently published trial, studying patients with AHFS and cardiorenal syndrome, showed that ultrafiltration was inferior compared to medical treatment, due to worsening renal function and due to more frequent adverse effects in the ultrafiltration group [290].
Consequently, there is currently no evidence favouring ultrafiltration over loop diuretics at all [290, 291]. Ultrafiltration, respectively renal replacement therapy, should be restricted to AHF patients who are: severely volume overloaded, staying oligo-anuric, despite all treatment efforts, are not responding to diuretic treatment, or in cases where acute severe kidney injury ensues [204].
7.5.2 BP/Renal Perfusion Pressure
Maintenance or restauration of a sufficient renal perfusion pressure, MAP (since the MAP best represents perfusion pressure [292]), is essential to preserve or re-establish renal function [118, 293–296].
Studies on mammalians revealed renal autoregulation to be working within a range of 80–180 mmHg [297–299]. Older study results examining the target MAP level in case of renal dysfunction, and even diuretic resistance associated with AHFS and other critical maladies are inconsistent [300–303]. However, more recent studies demonstrate that MAPs between 75 and 85 mmHg do not only enhance renal perfusion pressure as desired and necessary, but are obviously beneficial in addressing altered renal microcirculation [144, 304, 305]. Patients with afflicted renal microhemodynamics will probably benefit from MAPs ≥75 mmHg [305–307]. Furthermore, in patients with coronary artery disease and CS, MAPs between 70(75) and 80 mmHg are suggested in order to stabilize the circulatory conditions [303, 308, 309]. Moreover, once autoregulation has been lost, re-establishment is supposed to require higher MAPs [295, 302]. In the meantime, no concerns and no evidence have been found that noradrenaline (NA), the most advantageous and preferred vasopressor agent [310], may be associated with an increased risk of AKI [145, 302, 311–314], if the indication to apply NA is straightforward, to address arterial hypotension in life-threatening circumstances, shock states and vasodilatory conditions [310, 315–317]. Accordingly, a MAP of around 80 mmHg should be targeted, although in each patient treatment should be individualized [293, 307, 314, 318, 319].
7.5.3 Further Measures
Activation of the renin-angiotensin system allows maintenance of glomerular perfusion pressure and glomerular filtration rate, despite reductions in cardiac output and BP, through preferential constriction of the efferent glomerular arteriole in patients with HF [213]. By addressing the neurohormonal activation and thus affecting heart–kidney cross-talk (attenuating inflammation and endothelial dysfunction), fluid retention and vasoconstriction is blunted, and concurrently cardiac and renal function stabilizes [22, 34, 176, 320]. Further, ACE-inhibitors and angiotensin receptor blockers are key agents in the therapy of systolic heart failure (HFrEF) [321–325]. They may counteract or mitigate side-effects of the diuretic medication, potentially further triggering neuroendocrine activity [22]. However, blocking the effects of RAAS may impair autoregulation of GFR [326], as attenuation of angiotensin II effects cause glomerular efferent arteriole dilation with a subsequent drop in glomerular perfusion pressure, resulting in a lower GFR and an increase in serum creatinine [192, 219]. Moreover, there is scarce data about the role of ACE-inhibitors/angiotensin receptor blockers in CRS, and their application in this condition is more or less empirically and based on expert opinion [6, 107, 327]. If ACE-inhibitors/angiotensin receptor blockers are initiated in the presence of
- (a)
hypotension (MAP <60 mmHg), and/or
- (b)
LVEDP <15 mmHg, and/or
- (c)
hyponatremia, and/or
- (d)
high dosages of loop diuretics are given,
renal function may significantly worsen [328, 329]. Furthermore, timing to initiate ACE-inhibitors/angiotensin receptor blockers is unclear: Some authors recommend that treatment should not be initiated before the patient is stabilized [6, 107]. However, in patients with moderately diminished renal function (and with diuretic resistance), ACE-inhibitors/angiotensin receptor blockers are likely to be beneficial and to offer survival benefit, although renal function may transiently (further) worsen [327]. In case of severely impaired renal function, it is unknown if they are beneficial or deleterious [330]. Dosing should be cautiously carried out starting with low dosages and some clinicians tolerate reductions in GFR up to 30% [107, 331]. It may be advisable to reduce the dosages of diuretic drugs before starting up with ACE-inhibitors/angiotensin receptor blockers [331]. Drops in BP should be avoided [6], and in patients who were on ACE-inhibitors/angiotensin receptor blockers prior to cardiac decompensation, a transient dose reduction may be appropriate [332]. Nevertheless, ACE-inhibitors/angiotensin receptor blockers are underused and application even in CRS type1 needs to be encouraged [333].
For the treatment with β-blockers, it may be opportune to withhold them until the patient is hemodynamically stable, unless AMI is the underlying aetiology where low dosages may be beneficial [34, 107]. This is because β-blockers may attenuate necessary and initially beneficial compensatory effects of sympathetic nervous system and thus may contribute to the development of cardiogenic shock (CS) [34, 334].
Notable for practical issues: Mild increases in createnine during diuretic treatment may be interpret as transient intravascular volume depletion or “overdiuresis” (if so, continue less aggressive with lower doses) [207], and may further occur in those patients who are on ACE-inhibitors [328] or where BP is apparently too low [207]. However, study results suggest that some degree of createnine increase, associated with ACE-inhibitor therapy, should be tolerated (increase up to 30% of baseline) [107, 207].
Further, keep in mind, that via fluid retention (and associated elevated CVP and RA-P), a normal MAP could be achieved and preserved (successful compensation), but often at the cost of amplified congestion and oliguria (high renal venous pressure). Diuretics given in such circumstances may worsen the situation by inducing a drop in BP while simultaneously stimulating sodium and water reabsorption. Consecutively a vicious cyle may be established [82].
To summarize, the traditionally close relationship and interconnection between heart and kidney function has recently been termed cardiorenal syndrome, CRS [4]. The pathophysiology is multifactorial and complex, and the features causing renal malfunction in type 1 CRS may individually vary [4, 5, 7]. However, in type 1 CRS, diminished RBF and deficient renal perfusion pressure, increased intra-renal vascular resistance and enhanced renal venous pressure (concomitantly causing renal venous congestion), are the fundamental hemodynamic aberrations precipitating intra-renal and intraglomerular alterations, and thus determining the pathophysiology [36, 76, 97, 169, 174, 252]. “Systemic venous congestion” (and thus renal venous congestion) is acknowledged to be “the major driver of acute cardiorenal syndrome” in type 1 CRS [36, 76, 169, 174, 252]. Nevertheless, GFR remains stable unless renal autoregulation is attenuated or impaired. The latter may arise:
- 1.
in case of severe hypotension and/or markedly reduced CO resulting in hypoperfusion,
- 2.
when renal perfusion pressures are beyond the autoregulatory threshold, and/orStay updated, free articles. Join our Telegram channel
Full access? Get Clinical Tree
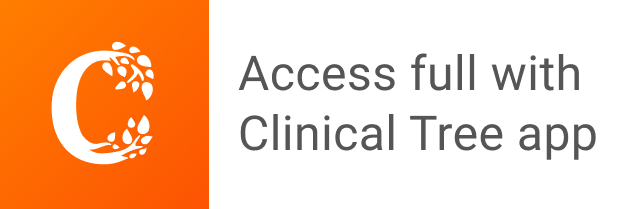