A 46-year-old man with a dilated cardiomyopathy and known chronic HFrEF for the last two years now presents with a recent history of worsening shortness of breath and fatigue with physical activity. His LVEF is 25% and he has enlargement of all four chambers of his heart on echocardiography. He was last hospitalized two months ago with fluid retention that responded to high dose intravenous diuretic therapy. He did not need an intravenous inotropic or vasodilator drug. He is taking maximally tolerated doses of carvedilol, sacubitril/valsartan, spironolactone, and bumetanide. He remains in sinus rhythm and has an ICD for primary prevention of sudden cardiac death. His QRS duration on his ECG is only 100 msec; therefore, he is not a candidate for chronic resynchronization therapy. Because of his worsening exercise tolerance, he is referred for a cardiopulmonary exercise test to evaluate his functional limitation and to assess his prognosis and candidacy for advanced heart failure therapies. He walked for 4 minutes on a treadmill following a modified Naughton protocol and stopped due to dyspnea and fatigue. He only attained 70% of his predicted maximal heart rate as he was taking a beta-blocker. There were occasional premature ventricular beats during exercise but no sustained arrhythmia. His oxygen saturation by pulse oximetry remained greater than 90% throughout exercise. He had a 10 mmHg decrease in systolic blood pressure at peak exercise. His heart rate recovery was abnormal with only a 5 bpm decrease at one minute of recovery during a slow walking cool-down period. His peak VO2 was 10.5 mL/kg/min which is 29% predicted for his age and gender. His peak respiratory exchange ratio (RER) was 1.20, indicating a near maximal effort. His VE/VCO2 slope was 48 and his oxygen uptake efficiency slope (OUES) was 1.1. He had evidence of oscillatory ventilation during exercise. His end-tidal CO2 (PETCO2) at rest was 28 mmHg and it decreased during exercise to 22 mmHg. The results of this test confirmed his severe exercise limitation and were consistent with diminished cardiac output and the development of pulmonary hypertension during exercise. In addition, the low peak VO2 , elevated VE/VCO2 slope, decreased OUES, abnormal PETCO2 response, oscillatory ventilation, exertional hypotension, and abnormal heart rate recovery all indicated that this patient had a poor prognosis with his HFrEF over the next year. A subsequent right heart catheterization confirmed the presence of a low resting cardiac index and pulmonary hypertension due to an elevated pulmonary capillary wedge pressure of 34 mmHg. His transpulmonary gradient was elevated at 15 mmHg but he had a favorable response to intravenous nitroprusside with a resulting pulmonary vascular resistance (PVR) of 2.1 Wood units. Based on these studies he was listed for cardiac transplantation. He subsequently required several hospitalizations for volume overload in the next few months and required inotropic therapy. He was unable to be weaned from milrinone and a left ventricular assist device was implanted as a bridge to transplantation as he had type O blood and his waiting time on the transplant list was expected to be prolonged.
Exercise intolerance is a hallmark of both chronic heart failure with reduced ejection fraction (HFrEF) and chronic heart failure with preserved ejection fraction (HFpEF). Patients complain of exertional dyspnea and fatigue and these symptoms relate to both elevated cardiac filling pressures and reduced cardiac output. In addition, there are abnormalities in the peripheral extraction of oxygen that limit exercise performance. It is plausible that patients with more severe heart failure (HF), both HFrEF and HFpEF, would have more functional limitation due to worsening of both cardiac and peripheral maladaptations. Thus, determination of exercise capacity becomes an important aspect of the care of these patients. It is important to realize that the syndrome of HF affects more than the cardiovascular system. There are abnormalities in the respiratory, skeletal muscle, and nervous system that play important roles in the regulation of cardiac and respiratory responses during exercise. Testing that incorporates an analysis of the interplay between these systems would be the ideal way to evaluate disease severity, categorize the degree of disability, and potentially assess prognosis in patients with HF. Routine treadmill testing has been used to evaluate exercise capacity in HF patients with some limited success in estimating functional capacity and assessing prognosis. This type of testing has limited value due to concerns about reproducibility and limited data about mechanisms responsible for the observed reduction in exercise capacity. In addition, exercise capacity as determined by an estimated metabolic equivalent (MET) level is often overestimated on these tests in HF and other cardiac patients. Cardiopulmonary exercise (CPX) testing has been recognized as a noninvasive tool to assess the interactions of the cardiovascular system with gas exchange physiology during exercise in normal subjects and patients with a variety of cardiovascular disorders. The American Heart Association and the European Association of Cardiovascular Prevention and Rehabilitation have both produced scientific statements describing the use and interpretation of these tests in a variety of clinical conditions, including HF.1,2 CPX testing in HF patients has several important benefits including determining whether exertional dyspnea is caused by HF, determining the severity of disease, providing important prognostic information that may lead to advanced HF therapy in HFrEF patients, determining the efficacy of new pharmacologic or device therapies, and creating an exercise prescription for cardiac rehabilitation.
The conduct of a CPX test is similar to a regular exercise test with the addition of expired gas (O2 and CO2) analysis and quantification of ventilation. Heart rate (HR), blood pressure (BP), electrocardiogram (ECG) recordings, and usually O2 saturation by pulse oximetry are obtained at rest, during exercise, and in early recovery. The patient also breathes through a mouthpiece or face mask in a closed system with a 1-way valve that allows room air for inspiration and the capture of all expired air for analysis. The expired air is analyzed with a metabolic cart. The basic components of the equipment contained in a metabolic cart in CPX testing are illustrated in Figure 22-1. Analyzers are used for the determination of expired O2 and CO2 and usually a pneumotachometer is used to determine ventilation. These data are used to determine oxygen consumption (VO2), carbon dioxide production (VCO2), and minute ventilation (VE). The VO2 and VCO2 are reported as both mL/min and mL/kg/min and VE is reported as L/min. From these directly measured factors a variety of derived (calculated) parameters can be obtained including the respiratory exchange ratio (RER), which is the ratio of VCO2 to VO2, oxygen pulse (VO2/HR), work rate (VO2/watts), and ventilatory parameters including the VE/VCO2 slope, the oxygen uptake efficiency slope (OUES), and end-tidal CO2 (PETCO2). Normal values are available to interpret these data in individual patients.3 Patients can exercise on either a treadmill or cycle ergometer but in nontrained individuals, there is a 10% to 20% lower peak VO2 on a cycle compared to a treadmill. Exercise testing protocols are usually less aggressive in HF patients with more gradual increases in workloads. Low-level protocols with 1-MET increments in workload, such as the Naughton protocol, are often used. Ramp testing with gradual increases in speed and grade on a treadmill or progressive increases in resistance on a cycle ergometer are becoming the standard and are replacing the step-wise increases in workload seen in various protocols such as modifications of the Bruce protocol and Balke protocol.
One may ask how respiratory measurements, such as VO2, relate to the cardiovascular system. In Figure 22-2, the Fick equation is presented. VO2 is the product of cardiac output (oxygen transport) and the arteriovenous (a-v) O2 content difference (oxygen extraction). Thus, the measurement of VO2 reflects both cardiovascular function (cardiac output and muscle blood flow) and the peripheral utilization of O2. Because cardiac output is the product of HR and stroke volume, the Fick equation can be modified to represent the relationship of VO2/HR (oxygen pulse) to both stroke volume and the a-v O2 content difference. During heavy and near maximal exercise, the O2 extraction is maximal and the O2 pulse serves as a good surrogate of the stroke volume response. Patients with chronic HF, both HFrEF and HFpEF, have both reduced cardiac output and altered O2 extraction during exercise. In one of the first clinical experiments using both CPX testing and invasive hemodynamic monitoring, Weber and colleagues demonstrated that the peak exercise VO2 was more closely related to the cardiac output response to exercise.4 Thus, in most patients with chronic HF the VO2 at peak exercise is a good surrogate for the cardiac output response. Similar studies performed in patients with HFpEF also show a strong relationship between VO2 and cardiac output, although there is more of a role for altered a-v O2 content difference in the determination of peak exercise VO2.
During progressive exercise there is a similar increase in both HR and VO2 up to maximally tolerated exercise (Figure 22-3). Thus, there is a direct relationship between HR and VO2 even in the presence of cardiac disease or pharmacologic therapy. However, the magnitude of the relationship can be altered. In patients with HF there is a reduction in the VO2 at a given workload and this will influence both the O2 pulse (VO2/HR) and the work rate (VO2/watt). The VO2 at maximally tolerated work is the gold standard for the description of aerobic exercise performance. If the VO2 value reaches a plateau despite an increase in workload, this is termed “VO2 max” (Figure 22-4). However, patients with cardiac disease are unable to attain such a high level of exertion; the VO2 attained in the last 15 seconds of exercise is termed the “peak VO2” and this value has been shown to be reproducible in HF patients. The peak RER is often used to determine if the patient has reached near maximal exercise capacity. A RER of 1.20 is considered near maximal capacity and a CPX test in a HF patient has good test-retest reproducibility if the RER is >1.05.5 The maximal or peak VO2 is influenced by age, gender, genetic disposition, training or deconditioning, environment, disease, and medications. In recovery there is a rapid decrease in both HR and VO2 and the rate of recovery is influenced by both parasympathetic activation and the rate of recovery of the oxygen deficit incurred during exercise. These responses are usually abnormal in HF patients with more prolonged rates of recovery. Heart rate recovery has been shown to have important clinical and prognostic value in HF patients. To date, evaluation of VO2 recovery has limited clinical utility in HF patients. The determination of VO2 during submaximal exercise also has important physiologic and clinical significance. The ventilatory threshold (VT) is the highest attained VO2 where there is still no significant accumulation of lactic acid in skeletal muscle and no switch to anaerobic metabolism. This threshold was formerly termed the anaerobic threshold, but anaerobic metabolism is not always present. At the VT there is an increase in ventilation to accommodate the increase in CO2 produced by the conversion of lactic acid to lactate. Thus, there is a change in the relationship between VO2 and ventilation but not between VCO2 and ventilation as ventilation increases to eliminate the excess CO2 produced in higher-intensity exercise. Physical activity above the VT will never reach a physiologic steady state and there will be progressive increases in HR, BP, sympathetic stimulation, and eventual cessation of exercise. The VT can be determined by various methods (Figures 22-5 and 22-6). The most reliable method is the V-slope method (Figure 22-5) where the relationship changes between VCO2 and VO2. The other represented method uses the ventilatory equivalents for VO2 and VCO2 (Figure 22-6). In this method the relationship between VE and VCO2 remains unchanged due to the increase in ventilation required to eliminate excessive CO2 while there is an increase in the VE and VO2 relationship. A third method (not shown) uses the end-tidal O2 and CO2 responses during exercise. The VT is reported as the VO2 at the threshold and is normalized as the percent of peak VO2. In healthy, untrained subjects, the VO2 at VT occurs at 45% to 65%. This measurement can be difficult to validate in HF patients due to a greater likelihood of a submaximal effort and ventilatory fluctuations that impede its determination. Patients with HF have been shown to have lower VO2 values at VT related to the severity of their illness and there has been some limited data indicating a worse prognosis with a lower VT.
Minute ventilation (VE) also increases progressively during exercise (Figure 22-7). In HF patients, VE at any given submaximal exercise workload is usually greater than in a normal subject while the peak exercise VE is lower due to a lower attainable workload. RER initially decreases early in exercise but then gradually increases through peak exercise (Figure 22-7). The RER is usually ≥1.10 at peak exercise and continues to increase due to the increase in VCO2 related to the respiratory removal of CO2. RER can be used as a determination of near maximal exercise capacity and is more reliable than the percent predicted maximal HR. However, a RER ≥1.1 should not be used as the determination for stopping a CPX test.
As noted earlier, patients with HF have a higher VE at submaximal exercise and a lower VE at peak exercise when compared to a healthy person (Figure 22-8). The decrease in peak exercise VE is related to a lower workload and subsequently lower peak VO2 than in a healthy subject. However, the higher VE during submaximal exercise represents excessive ventilation during exercise that is commonly seen in HF, both HFrEF and HFpEF. This increase in ventilation is a result of several mechanisms that influence ventilatory drive (Figure 22-9). With progressive HF there is an increase in cardiac filling pressures (LV end-diastolic pressure and pulmonary capillary wedge pressure) and a decrease in cardiac output/index. These hemodynamic alterations result in an increase in ventilation/perfusion mismatch in the lungs with adequate ventilation but poor perfusion. In addition, there is an increase in chemoreceptor and ergoreceptor sensitivity and activation that results in an increase in the rate and depth of breathing. Thus, there is excessive ventilation for any given VCO2 during exercise. This relationship has been described throughout exercise, at the VT, and at peak exercise. The most common description of this relationship is the linear relationship between VE and VCO2 throughout exercise, described as the VE/VCO2 slope. This slope is not influenced by the mode of exercise or the aggressiveness of the exercise protocol and does not require near-maximal exercise. It can be obtained during submaximal exercise in most patients and is highly reproducible with repeated testing in a given subject. This relationship was reported to be elevated with prognostic significance in systolic HF patients.6 A normal VE/VCO2 slope is shown in Figure 22-10. In Figure 22-11, a HF patient has an abnormally elevated VE/VCO2 slope of 42.5. In both examples it is clear that there is a very statistically significant linear relationship between VE and VCO2, with the HF patient having a much steeper slope, indicating excessive or inefficient ventilation during exercise. A normal VE/VCO2 is <30. Patients with both HFrEF and HFpEF can have mild (30.0-35.9), moderate (36.0-44.8), and severe (≥45) excessive ventilation as represented by VE/VCO2. Values greater than 34 to 36 have been associated with worse prognosis in HFrEF patients.6 Patients with HFpEF can also have elevated VE/VCO2 slopes but the values are usually less than in patients with HFrEF. It is important to note that patients with pulmonary hypertension (HTN) and chronic lung disease can also have elevated VE/VCO2 slopes and by itself, an elevated VE/VCO2 slope during exercise is not diagnostic for HF. In patients with very elevated VE/VCO2 slopes >50, pulmonary disease alone or a mixed picture of HF and pulmonary disease should be considered.
The relationship between VO2 and VE during exercise is more complex. Because ventilation progresses at a more rapid rate than VO2 during exercise, the relationship is logarithmic (Figure 22-12). However, this relationship becomes linear when the log transformation of VE is related to VO2 (Figure 22-13). This relationship has been designated as the oxygen uptake efficiency slope (OUES). It represents the rate of increase in VO2 in response to a given VE during incremental exercise. Similar to the VE/VCO2 slope, this parameter can be obtained during submaximal exercise and a near-maximal exercise effort is not required. The OUES has been reported to be a measurement of the efficiency of O2 extraction and uptake. It is influenced by muscle mass, oxygen extraction and utilization, the level of PaCO2 set point during exercise, physiologic dead space in the lung with ventilation/perfusion mismatch, and the onset of lactic acidosis. More than VE/VCO2, the OUES incorporates cardiovascular, musculoskeletal, and respiratory function in a single index. As opposed to VE/VCO2, a lower OUES is more abnormal as it indicates more ventilation being required to consume the same amount of VO2. The OUES declines with age and is affected by lean body mass. In a study of normal subjects and patients with cardiac disease, the normal values for OUES were determined to be as follows: 1,175 – (15.8 × age) + (841 × body surface area) in women and 1,320 – (26.7 × age) + (1,394 × body surface area) in men.7 OUES has also been shown to have additional prognostic value when compared to peak VO2 and VE/VCO2 slope in chronic HF patients with a value <1.47.8 In Figure 22-14, there is a comparison between the OUES in a healthy subject and a patient with chronic HFrEF. The nonlogarithmic relationships are shown. The slope of the HF patient is substantially lower (23%) compared to that in the normal subject and it falls in the poor prognostic category. This patient went on to have a heart transplant. Despite its physiologic and potential clinical relevance, the OUES is rarely used alone to determine prognosis and therapy. Its use is limited by a few studies with limited numbers of patients, the lack of standardization of units, an unclear understanding of the most important physiologic mechanism explaining the relationship, the influence of body weight on the measurement, and the questionable additional prognostic value to other CPX variables. In addition, the OUES can also be abnormal in patients with pulmonary disease; however, there is less of an abnormality in pulmonary disease and a severely low OUES may discriminate between HF and lung disease.
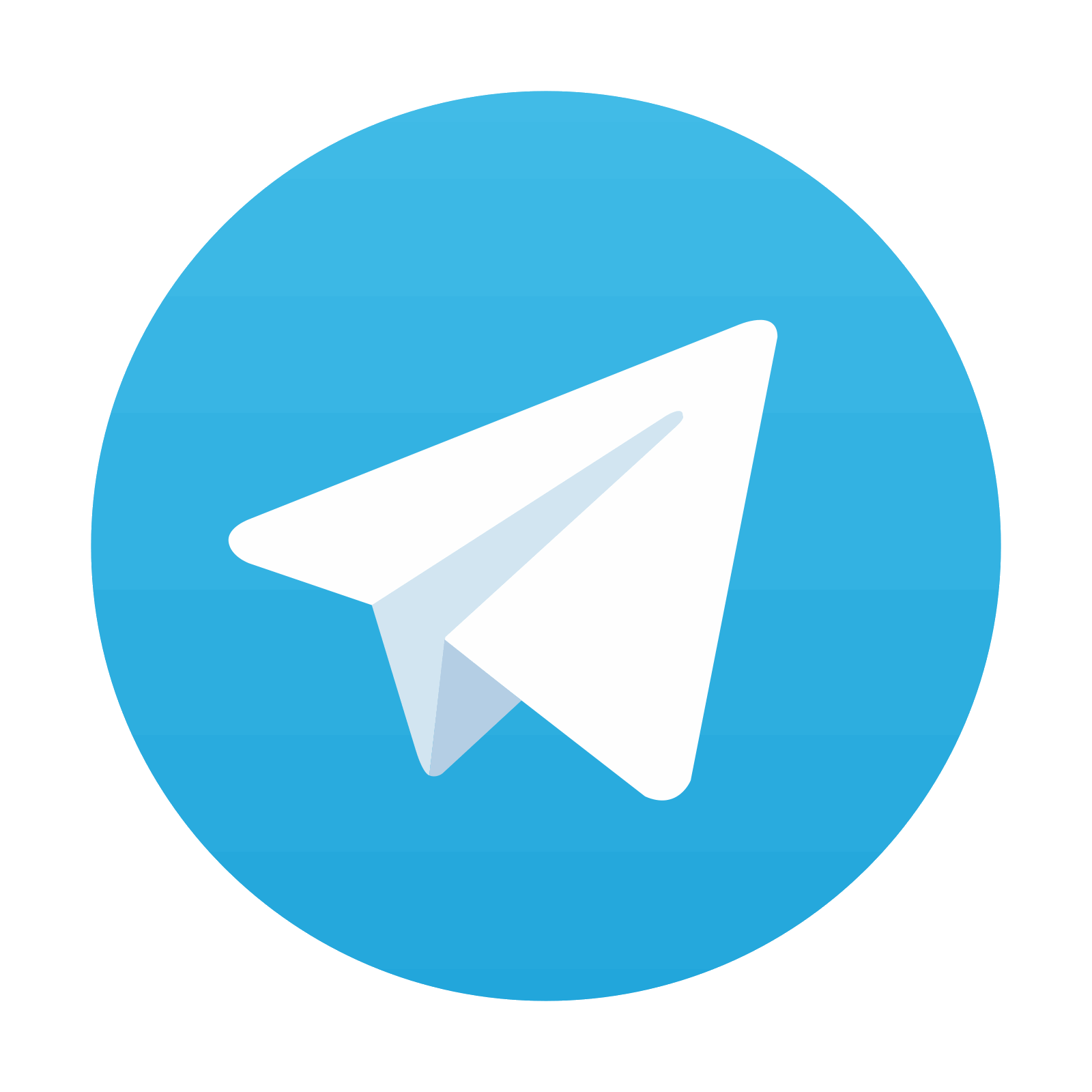
Stay updated, free articles. Join our Telegram channel
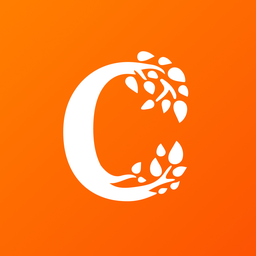
Full access? Get Clinical Tree
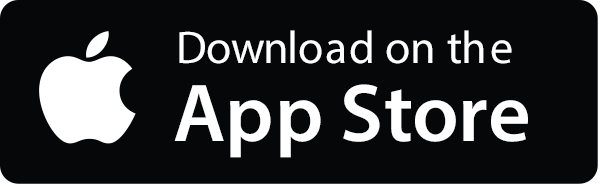
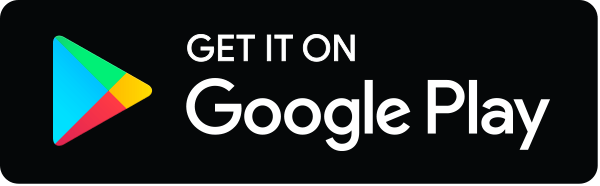