Regular exercise is an important part of a healthy lifestyle. Physical activity in some westernised societies has diminished for a variety of reasons as society has trended away from outdoor activities towards other types of activities, including computer games and cable television. Healthy levels of physical fitness require regular participation in activities that generate energy expenditures significantly above the resting level. 1
Exercise testing has become an integral part of the evaluation of children with congenital and acquired cardiovascular disease. Although children are seldom sitting or lying quietly during waking hours, most of the testing available to the cardiovascular specialist is performed in such a resting state, and sometimes in a sedated state. The evaluation of the patient in the exercise physiology laboratory allows the clinician to assess the cardiovascular system in a state that is more likely to be reflective of the normal daily physical activities.
Optimal exercise performance requires a continuous meshing of multiple systems of organs. 2 The roles of the heart and lungs are to provide adequate energy substrates to working skeletal muscle, and to remove the end products of aerobic and anaerobic metabolism during exercise. Most of the children that we encounter in the exercise physiology laboratory have, or are suspected of having, cardiac anomalies. Abnormalities of non-cardiovascular origin, however, may also play a role in limiting exercise performance in patients with surgically repaired complex congenital cardiac defects. Any malfunctioning of the muscles, bones, or nervous system will impair exercise performance. A modern laboratory for exercise physiology, therefore, must be capable of assessing all exercise-related systems, including the cardiovascular, pulmonary, neurologic, and musculoskeletal systems. Furthermore, accurate interpretation of exercise tests requires the proper equipment and personnel. This is particularly important as the cardiologist supervising the laboratory frequently evaluates children with real or suspected exercise limitations. These children seldom have any cardiovascular abnormalities, but may have involvement of other organs, such as dysfunction of the pulmonary or musculoskeletal systems. Properly performed exercise testing, and its interpretation, should also be able to identify or exclude abnormalities of extracardiac organs that normally contribute to exercise performance.
In this chapter, we review briefly basic exercise physiology as it relates to cardiopulmonary exercise testing. We discuss the equipment required for performing an exercise test, and its applications, assessing the types of data obtained from exercise testing, and their usefulness in the evaluation of the child with cardiovascular disease. We also review indications and contraindications for exercise testing in cardiovascular disease.
BASIC EXERCISE PHYSIOLOGY
Assuring proper exercise performance requires the seamless and continuous meshing of multiple systems of organs. 3,4 The classic illustration of Wasserman and colleagues ( Fig. 20-1 ) is the widely duplicated figure that depicts the interaction of the key systems that couple internal cellular respiration to external pulmonary respiration during exercise. It shows the systems as a series of overlapping cogs, all of which must mesh seamlessly to allow exercise to occur. In such fashion, mechanical energy is produced from chemical energy at the cellular level, with subsequent delivery and removal of substrates for energy production and byproducts of muscle metabolism. All of this is accomplished while maintaining chemical and thermal homeostasis within narrow ranges.

CELLULAR METABOLISM
Adenosine triphosphate is the chief source of chemical energy for the exercising muscle. Mechanical energy is produced by the process of excitation-contraction coupling, driven by the breakdown of adenosine triphosphate and the release of inorganic phosphates and adenosine nucleotides. The stores of adenosine triphosphate and phosphocreatine in the myocytes are sufficient only for 10 to 15 seconds of activity, and so must continuously be replenished. As can be seen in Figure 20-2 , adenosine triphosphate is produced in small amounts in the cytosol by anaerobic metabolism. Much larger amounts are produced aerobically in the mitochondria. 3,5

Anaerobic and aerobic metabolic activities use glucose, which is metabolised to pyruvate. Pyruvate then has two possible fates. It may be converted into lactic acid, and excreted into the bloodstream, where it is buffered by sodium bicarbonate converting it to lactate. This reaction results in the production of carbon dioxide and water, along with small amounts of adenosine triphosphate, and the former are excreted in the lungs. The lactate molecule is taken up by the liver for resynthesis to glucose and glycogen, which can then be utilised again for energy production.
The other fate of pyruvate is aerobic metabolism. Pyruvate is converted into acetyl-coenzyme A and transported into the mitochondria where it enters the Krebs cycle, again producing carbon dioxide and water. Adenosine triphosphate is produced in large quantities via the electron transport chain, with oxygen functioning as the terminal electron acceptor. Unlike anaerobic metabolism, fats as well as carbohydrates and proteins can undergo aerobic metabolism. Fats enter aerobic metabolism at the level of the Krebs cycle, and do not undergo anaerobic metabolism. 4,5
During any activity, the availability and use of substrates, primarily fats or carbohydrates, will vary depending upon the type, intensity, and duration of activity. Fats are more reduced than carbohydrates, requiring more oxygen for complete oxidation compared to carbohydrates on a mole for mole basis. 4 The ratio of production of carbon dioxide to consumption of oxygen, abbreviated to Vco 2 /Vo 2, is called the ratio of respiratory exchange, or if in a steady state, the respiratory quotient. In a state of high use of fat, the ratio is approximately 0.7. Conversely, during pure carbohydrate metabolism, the ratio is 1.0, reflecting the lower amount of oxygen needed to oxidise carbohydrates. The stores of glycogen in the adult body are seldom more than approximately 1500 Kcal. Some use of fat, therefore, is almost always required. As a result, the resting ratio of respiratory exchange, even in the well-fed state, will usually range from 0.8 to 0.9.
During a typical graded maximal exercise test, the work rate is gradually increased over the course of 10 to 15 minutes, as explained in our subsequent sections concerning exercise protocols. Production of adenosine triphosphate will need to increase as mechanical work increases, and at low levels of work this increase is met predominately by increased aerobic metabolism. Slow twitch muscle fibers, so-called Type 1, with high oxidative metabolism, are primarily recruited for this activity. As work rate increases, consumption of oxygen increases in a linear fashion ( Fig. 20-3 ). Near peak work rates, consumption will tend to plateau, as maximal consumption is achieved. This phenomenon is often absent in children. 4,6,7

As consumption of oxygen increases in response to increased work rate, there is a gradual rise in the ratio of respiratory exchange. The reason for this rise is twofold. First, there is a gradual shift in use of fats compared to carbohydrates. This shift allows for more efficient use of oxygen, as the yield of adenosine triphosphate per mole of oxygen is greater with carbohydrates. Secondly, the rise in the ratio occurs as a result of increased levels of lactate in the blood. At approximately 50% to 60% of the maximal consumption of oxygen, levels of lactate begin to rise in the serum. The point where clearance can no longer keep up with production is known as the lactate threshold. The onset of anaerobic metabolism by the exercising muscles is responsible for this production of lactate. Above the threshold, levels of lactate rise exponentially as work rate increases, necessitating increased buffering by sodium bicarbonate in order to maintain blood pH homeostasis. The byproduct of the buffering process, carbon dioxide , must be removed in order to maintain levels within the normal range. This causes a significant rise in production of carbon dioxide out of proportion to the rise in consumption of oxygen, resulting in the respiratory exchange ratio increasing to greater than 1.0. The ratio in adults at peak exercise may be as high as 1.2 to 1.4, but is usually somewhat lower in children. 6–11
The increase in production of carbon dioxide associated with the buffering of lactate allows for measurement of a non-invasive surrogate of the lactate threshold. This surrogate, known as the ventilatory anaerobic threshold, is defined as the point where production of carbon dioxide, and minute ventilation, begin to rise out of proportion to the consumption of oxygen. Like the lactate threshold, the ventilatory anaerobic threshold occurs at approximately 50% to 60% of the maximal consumption of oxygen. It may be significantly lower or higher in de-conditioned or highly trained athletes, respectively. Like maximal consumption of oxygen, the ventilatory anaerobic threshold is a physiological limit, and has been used as a marker of aerobic fitness. Unlike maximal consumption of oxygen , the ventilatory anaerobic threshold has the virtue of being effort independent, is a level of exercise that can be sustained over a prolonged period of time, and is easily affected by training or sedentary behavior. Unfortunately, the ventilatory anaerobic threshold can be difficult to measure accurately in smaller children who tend to have erratic patterns of breathing. 6–11
THE HEART AND LUNG AS SERVICE ORGANS
As previously mentioned, consumption of oxygen rises in near linear response to increasing work rate. The consumption can be derived from the Fick equation as follows:
Vo 2 = Q × ( a − v O 2 diff .)
where Vo 2 is consumption, Q is cardiac output, and a − v O 2 diff. is the difference in content of oxygen is arterial and mixed venous blood. Q is defined as:
Q = SV × HR
where SV is stroke volume and HR is heart rate. Rearranging the equations, we see that Vo 2 is:
Vo 2 = SV × HR × ( a − v o 2 diff . )
The rise in consumption of oxygen during exercise, therefore, is dependent on the increases in stroke volume and heart rate, and widening of the difference in the content of oxygen in arterial and mixed venous blood. During strenuous exercise, cardiac output may rise as much as fivefold over resting levels. 5,12–15 Both stroke volume and heart rate contribute to this increase, but the relative contributions of each are different. 5 At rest, stroke volume is approximately 60% of its maximal value. At the onset of exercise, a combination of increased venous return and sympathetic tone causes stroke volume to increase. This occurs as consequence of two mechanisms. First, increased preload stretches the myocyte and increases tension, resulting in changes in the Frank–Starling forces. Second, the increase in sympathetic tone results in an increase in the inotropic state of the myocardium. This increase in inotropy improves the active tension developed for any given preload, thus further augmenting stroke volume. Most of the increase in stroke volume takes place below 30% to 40% of the maximal consumption of oxygen ( Fig. 20-4 ). At higher heart rates diastolic filling time is decreased, which limits any further augmentation in stroke volume irrespective of any increase in the inotropic state. Therefore, at higher heart rates, and naturally at higher work loads, the relative contribution of stroke volume to the overall increase in cardiac output is small.

An increase in heart rate is the primary mechanism for the increased cardiac output at higher work rates. This is reflected in the essentially linear relationship between heart rate and consumption of oxygen as work rates increase (see Fig. 20-4 ). The ability to increase heart rate in a normal manner during exercise, therefore, is essential to achieving a normal aerobic workload. The difference in content of oxygen between the arterial and mixed venous blood gradually widens with increasing work rate and consumption of oxygen, as a result of increased extraction of oxygen by the exercising muscles. The byproducts of myocytic metabolism, including hydrogen, carbon dioxide, and lactate, all lower the pH, thereby shifting the oxygen-haemoglobin dissociation curve rightward, favouring increased unloading of oxygen at the level of the exercising muscle. Such unloading is more pronounced at higher intensities of exercise, when the concentration of metabolic byproducts is greater. This will be discussed in more detail below in the relationship between consumption of oxygen and cardiac output.
DISTRIBUTION OF BLOOD FLOW
For consumption of oxygen to increase during exercise, it is essential that cardiac output not only increase, but that blood flow is preferentially shunted to the exercising muscles ( Fig. 20-5 ). At peak exercise, blood flow to exercising muscle may be 80% or more of the total cardiac output. The redistribution of blood flow is achieved by a combination of autonomic and metabolic vasoregulatory mechanisms. 16 Exercise is essentially a state of increased sympathetic nervous system tone, which is regionally overridden by metabolic vasodilation. The increase in sympathetic tone associated with exercise results in a generalised constriction of the precapillary resistance arterioles. Simultaneously, the sympathetic tone increases heart rate and cardiac contractility, resulting in increased cardiac output. 16–18 Vasodilation occurs at the level of the exercising muscle as a result of local metabolic changes. Aerobic and anaerobic metabolism, excitation-contraction coupling, and breakdown of adenosine triphosphate all result in the release of potent vasodilators into the interstitial spaces. These vasodilators include free potassium and hydrogen ions, carbon dioxide, lactate, adenosine diphosphate, and inorganic phosphate. The result is a profound vasodilation of the vascular bed in the exercising muscles.

Vasoconstriction of the splanchnic vascular bed results in either no change, or a decrease, in flow of blood to the gut and kidneys. The effect on the overall peripheral vascular resistance depends on the size of the exercising muscle groups. During dynamic exercise, which utilises large muscle groups, such as running, the net result is a significant drop in systemic vascular resistance, despite an overall increase in sympathetic tone.
SURROGATES OF CARDIAC OUTPUT
Exercise testing is frequently performed in order to assess the ability of the cardiovascular system to increase cardiac output in response to an increased workload. Measurement of cardiac output during exercise, however, is often not practical. Direct measurements are too invasive to allow adequate levels of exertion. Non-invasive methods are often technically difficult, and may be inaccurate in the pathophysiological states that are encountered in many types of congenitally malformed hearts. The obstacles accurately and reliably to measure cardiac output has lead to the use of consumption of oxygen as a surrogate of cardiac output in many clinical and research settings. 4,16,19 Over a broad range of consumption of oxygen there is a nearly linear relationship between consumption of oxygen and cardiac output ( Fig. 20-6 ).

Despite a plateau in cardiac output at very high work loads, consumption of oxygen continues to increase as a result of increased oxygen extraction, thereby increasing the arterial-mixed venous content of oxygen difference. Consumption of oxygen is determined by the amount of oxygen delivered in the blood that is extracted by the metabolically active tissues. During exercise, this extraction is determined by the myoglobin content of the exercising muscle, the isoenzyme characteristics of the muscle, as well as the physiological milieu in which the muscle is working. 4,16 The physiological state will have a great impact on the oxygen-haemoglobin dissociation curve. As previously stated, there are multiple byproducts of muscle metabolism which are released into the intercellular space during exercise, shifting the oxygen-haemoglobin dissociation curve to the right. 4 The local increase in muscle temperature during exercise also has the same effect. The net result is an increase in oxygen unloading to the exercising muscle particularly at high workloads. There is therefore an increase in the arterial-mixed venous content of oxygen difference, and a continued rise in consumption of oxygen, even in the presence of a flattening or plateauing of the rise in cardiac output near peak exercise. 4,16,17
Gender differences are present in the relationship between cardiac output and consumption of oxygen (see Fig. 20-6 ). Females tend to have a somewhat higher cardiac output for any given consumption, as well as a lower maximal consumption. 17 These differences are likely due to the difference in content of haemoglobin between males and females. Females have lower levels of haemoglobin in the serum compared to males, and therefore have lower contents of oxygen, necessitating a higher cardiac output to deliver an equivalent consumption of oxygen. Lower values in adolescent and adult females manifest clinically in slightly higher cardiac outputs, and lower consumption of oxygen at peak exercise. It is easy to comprehend how anaemia will result in reduced consumption of oxygen and a higher ratio of cardiac output to consumption of oxygen compared to non-anaemic states. The use of consumption of oxygen as a surrogate for cardiac output, therefore, is problematic in the presence of anaemia.
OXYGEN PULSE
In a clinical setting, the practitioner is frequently interested in assessing the intrinsic inotropic state of the myocardium during exercise. As was stated earlier, this state is primarily reflected by the stroke volume of the heart. A non-invasive measurement of stroke volume is a useful tool to assess myocardial function. This has given rise to the use of oxygen pulse during exercise testing. 20
The oxygen pulse is defined as:
Oxygen pulse = Vo 2 in mL / min / HR
Since Vo 2 = (SV × HR) × (a − v O 2 diff), the pulse also equals:
SV × ( a − v O 2 diff )
The oxygen pulse, therefore, is a value that can be measured at any given work rate or consumption of oxygen during exercise testing. A rise or fall in stroke volume will correlate with a rise or fall in the oxygen pulse. These changes can be blunted somewhat by an increase or decrease in the difference in content of oxygen between arterial and mixed venous blood, but the ability of muscles to extract oxygen usually does not significantly change except in response to prolonged training. This is particularly true at maximal exercise. The oxygen pulse is perhaps most useful in assessing changes in myocardial performance over time, or following therapeutic interventions in an individual patient. Assuming no change in the haemoglobin content, the content of oxygen of the arterial blood should be unchanged. Absent large weight changes, the consumption of oxygen required to perform a given amount of work on an ergometer for an individual patient is constant. Any increase in the oxygen pulse measured at a given work rate, therefore, would reflect a lower heart rate needed to achieve the same consumption of oxygen, and be indicative of a higher stroke volume. The converse would be true for a falling oxygen pulse. This does presume the haemoglobin content of the blood has not changed, and that the chronotropic state of the heart is also unchanged. A significant change in either of these limits the usefulness of the oxygen pulse to act as a marker of stroke volume during exercise.
PULMONARY RESPONSE TO EXERCISE
Consumption of oxygen, and removal of carbon dioxide, require that the cardiovascular and pulmonary systems work together as a single integrated unit. The ultimate goal is that for a given consumption of oxygen, minute carbon dioxide is eliminated while maintaining Paco 2 and pH within a narrow physiological range. For this reason, it is not surprising that there is a very tight relationship between minute ventilation, consumption of oxygen, and production of carbon dioxide. Such a relationship is demonstrated in ventilatory equivalents for carbon dioxide and oxygen, namely VE/Vco 2 , and VE/Vo 2 . The typical relationship of minute ventilation to increasing work rate, and the relationship of the ventilatory equivalents for carbon dioxide and oxygen to work rate, are depicted in Figure 20-7 . Note that there is a steady rise in minute ventilation with increasing work rate. Both ventilatory equivalents initially fall at the onset of exercise and then plateau. During the plateau phase, minute ventilation is increasing in proportion to both the increases in the ventilatory equivalents for carbon dioxide and oxygen. At the onset of the ventilatory anaerobic threshold, minute ventilation begins to increase out of proportion to consumption of oxygen as the respiratory drive is stimulated by increased production of carbon dioxide occurring as a consequence of the buffering of lactic acid. Note in Figure 20-7 B that, at this point, the ventilatory equivalent for oxygen begins to rise while the ventilatory equivalent for carbon dioxide remains stable. At maximal exercise, production of lactic acid may rise to a level that can no longer be adequately buffered by sodium bicarbonate and frank metabolic acidosis ensues. The normal physiological response to acidosis is a marked hyperventilatory response referred to as terminal hyperventilation . At terminal hyperventilation, both the ventilatory equivalents for oxygen and carbon dioxide rise, resulting in a significant fall in Paco 2 4,8–10

Minute ventilation is defined as:
VE = V T × F
where V T is tidal volume and F is respiratory rate.
At the onset of exercise, minute ventilation initially increases primarily by an increase in tidal volume rather than respiratory rate. Tidal volume includes both alveolar ventilation as well as the physiological dead space. Physiological dead space is made up of both anatomic components, the trachea and bronchi, as well as the functional dead space, namely the ventilation of hypoperfused or non-perfused pulmonary components. At rest the ratio of dead space to tidal volume is approximately 30% to 35% in adults and adolescents. With the increase in tidal volume at the onset of exercise, the ratio falls. This is due to a larger tidal volume relative to the fixed anatomic dead space. Additionally, there is an improvement in ventilation-to-perfusion matching as the increased negative thoracic pressure causes recruitment of additional capillary beds, resulting in a fall in the physiological dead space. At peak exercise, the ratio of dead space to tidal volume may fall to approximately 5% to 15%. Children typically have less efficient ventilation than either adolescents or adults, and tend to have a higher respiratory rate for any given minute ventilation, resulting in a higher ratio at any level of exercise. 21–25
At higher levels of exercise, minute ventilation increases in terms of both tidal volume and respiratory rate. Much of the improvement in the ratio occurs at lower to moderate levels of work. The rapid fall in the ratio is responsible for the initial steep fall in the ventilatory equivalents for oxygen and carbon dioxide that occurs at the onset of exercise (see Fig. 20-7 B). Although both consumption of oxygen and production of carbon dioxide are increasing in early exercise, this is more than compensated for by the improved efficiency of the lungs, resulting in a less than proportional rise in minute ventilation.
In healthy children and adults, ventilation is not the rate-limiting step in exercise performance. 4,20 This is because the cardiovascular system usually reaches its limit of delivery of oxygen before the pulmonary system is exhausted. At the maximal level of exercise, most subjects have not maximally stressed their pulmonary system, and there is pulmonary reserve, which is described by exercise physiologists as the breathing reserve. This is the theoretical ability to increase minute ventilation that remains untapped at maximal minute consumption of oxygen. This value is in the range of 20% to 50% in healthy children and adults. 20
The breathing reserve is defined as:
( 1 − max VE / MVV ) × 100
and is expressed as a percentage, where VE is the maximally achieved VE at peak exercise and MVV is maximal voluntary ventilation.
Maximal voluntary ventilation is obtained by having the subject hyperventilate for 10 seconds as vigorously as possible prior to exercise testing. Much longer periods of rapid and deep breathing may result in fainting, and should be avoided. The volume achieved is then multiplied by six, theoretically to give a maximally achievable minute ventilation. A low breathing reserve, of less than 20%, may indicate that primary pulmonary abnormality is limiting exercise performance. As maximal voluntary ventilation is a highly effort dependent measurement, care must be taken when interpreting breathing reserve. Other confirmatory data, such as an abnormal response of the ventilatory equivalents, or abnormal resting spirometry, should be sought to confirm pulmonary abnormalities.
LABORATORY REQUIREMENTS
Environment
Adequate space and environmental controls are important in order to assure a successful exercise test. The child should be made to feel comfortable and relaxed, which will improve exercise performance. Sufficient space is needed to accommodate the various ergometers and monitoring equipment, including emergency resuscitation equipment, while maintaining adequate space to access the patient in emergency situations. A minimum of 250 square feet of space is required, with 500 square feet or more when multiple work stations are employed. 26
The climate of the laboratory must be well controlled to allow proper thermoregulation during the exercise test. The room should be well ventilated and temperatures should be regulated between 20° C and 23° C, 26,27 a temperature range which permits the child to be comfortable at rest but still allows for adequate dissipation of heat during exercise. Humidity should be approximately 50% to ensure free perspiration during exercise. 27
The laboratory should be child-friendly. Television monitors with appropriate programming, and wall posters, have proven useful in minimising anxiety and/or boredom of the patients.
Safety Precautions
Exercise testing has been performed in children with very low risk, even in those who have complex cardiovascular disease. 28,29 Although significant complications of exercise testing are rare, proper safety precautions are essential. Key staff usually include at least one physician who is well trained in paediatric exercise testing. A physician does not need to be present for testing patients deemed to be at low risk or healthy, 27,30 but should be present at the testing of any child deemed to be at increased risk of a complication, such as a child with a life-threatening arrhythmia or syncope. The American Heart Association published guidelines for patients who are at low risk for exercise complications, and require a physician available, but not physically present ( Table 20-1 ). 30 The risk for each patient must be individually assessed. Ideally, two staff members trained in exercise testing should be present for all tests. All exercise physiologists or exercise technicians should be familiar with paediatric exercise testing, and at least one of these personnel should be trained in paediatric advanced life support. A well-stocked emergency resuscitation cart, defibrillator, system for delivery of oxygen, and suction apparatus are also essential. 30
|
Preparation of Patients
Education and preparation of the children before and during their test are probably the most important factors in successful testing. A thorough explanation of the testing procedure results in better compliance and effort, especially in younger children. Children should avoid heavy meals for approximately 2 hours prior to the test, and wear appropriate clothing, such as shorts, T-shirts, and athletic shoes. Proper preparation of the skin helps to ensure an adequate electrocardiographic signal. 31 Superficial abrasions should be performed to remove the top layer of epidermis and enhance the electrical signal. Electrodes should be large enough to provide a good contact with the skin.
The patient should be instructed in the procedures to be used during the exercise test. These include proper use of the treadmill or cycle ergometer, and the use of hand signals to convey the level of fatigue, distress, or symptoms. Scales of perceived exertion, such as the Borg scale, can be useful in communicating with the child during the study. Such scales allow those conducting the testing to estimate when the child is likely to reach maximal effort ( Fig. 20-8 ). 32 Special attention should be given to maneuvers, such as spirometry or inert gas rebreathing, that require proper technique and cooperation from the patient.
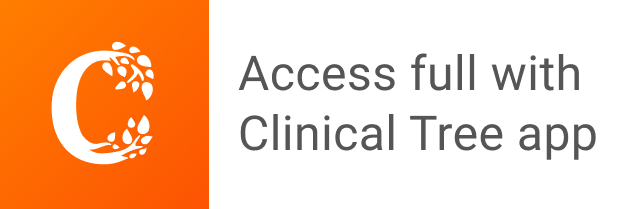