Abstract
Over the past two decades survival from pediatric cardiac arrest has improved dramatically, particularly in the in-hospital setting, from 15% to nearly 50%. The reasons for this are multifactorial and include but are likely not limited to the use of ECPR, personalizing the care delivered to the child’s age and also the child’s underlying cardiac anatomy and physiology, and applying a multitude of approaches to improving the quality of care we deliver. Teams are now systematically addressing the quality of cardiopulmonary resuscitation (CPR) delivered during the event with real-time feedback with bedside devices and quality CPR coaches, postevent debriefing, and meticulously managing the post–cardiac arrest care period. Unfortunately, there is evidence of great variability between hospitals, suggesting there are still lives to be saved. In the upcoming decades we need to continue to explore physiologic targets to optimize coronary and cerebral perfusion while minimizing harm from compressions, use human factors to improve team dynamics and room ergonomics, refine approaches to post–cardiac arrest care, and improve our ability to prognosticate on outcomes. Although avoiding a cardiac arrest is always best, we now know that many lives can be saved with exquisite CPR, and we must continue to focus on optimizing outcomes for these most vulnerable of patients.
Key Words
cardiac arrest, cardiopulmonary resuscitation (CPR), extracorporeal CPR (ECPR), chest compressions, defibrillation
Cardiac arrest is a relatively rare phenomenon in children. Although the overall incidence is rare, cardiac arrest represents a clinically important event often resulting in death or poor neurologic outcome. Out-of-hospital cardiac arrest (OHCA) is estimated to occur approximately 16,000 times per year (8 to 20 per 100,000 children annually) in the United States. In-hospital cardiac arrest (IHCA) has been variably estimated to occur in 5000 to 10,000 children per year, or in 0.77 per 1000 admissions (or 77 per 100,000 children). Thus IHCA occurs at least four times more frequently than OHCA. Higher survival to discharge after in-hospital compared with out-of-hospital arrest rates have been attributed to differences in cause of arrest and more rapid recognition and treatment by skilled caregivers in the in-hospital setting. Among hospitalized children, cardiac arrest is reported in 2% to 4% of all children admitted to a pediatric intensive care unit (ICU) and in approximately 3% to 6% of children admitted to a cardiac ICU. Cardiopulmonary resuscitation (CPR) is performed in 7 per 1000 hospitalizations of children with acquired and congenital cardiovascular disease, a rate greater than 10-fold that observed among hospitalized children without cardiovascular disease. Recently published data from the Pediatric Cardiac Critical Care Consortium (PC 4 ) demonstrated a cardiac arrest rate of 3.1% among 15,908 cardiac ICU encounters (6498 medical and 9410 surgical) in 23 centers. Observed (unadjusted) cardiac ICU cardiac arrest prevalence varied from 1% to 5.5% with wide variation in cardiac arrest rates per 1000 cardiac ICU days among the 23 centers (1.1 to 10.4).
Survival from IHCA in infants and children has significantly improved over the past four decades, from approximately 9% in the 1980s to at least 14.3% in 2000, and most recent data reports overall survival has increased nearly threefold during the past decade to 43%. This significant improvement in survival is despite an increase in cardiac arrests resulting from nonshockable rhythms. These improvements have been facilitated by improvements in systems and processes to prevent cardiac arrest and improvements in CPR quality, resulting in higher rates of survival during the acute resuscitation period. Notably, these improved survival rates were not accompanied by increased rates of significant neurologic disability among survivors. A number of factors have likely played an important role in achieving these trends. First, clinical practice guidelines over the past decade have emphasized several aspects of the acute resuscitation chain of survival. These include greater vigilance and closer monitoring, which may have resulted in shorter response times. In addition, recent pediatric in-hospital publications have demonstrated a higher proportion of patients located in a monitored unit or an ICU at the time of cardiac arrest, which may have allowed earlier recognition of cardiopulmonary compromise and prompt initiation of resuscitation efforts.
Additionally, patients located in an ICU may have patient management/interventions in place at the time of the arrest (e.g., central venous and arterial access, improved cardiac monitoring) and/or the presence of team members experienced with resuscitation of the cardiac patient, which may contribute to improved survival after cardiac arrest. Additionally, specialized cardiac arrest processes, including easier access to extracorporeal life support during resuscitation (extracorporeal CPR [ECPR]), may improve survival after cardiac arrest when it occurs in the ICU. Increased participation in hospital-specific quality improvement efforts like the American Heart Association’s (AHA’s) Get With the Guidelines—Resuscitation (GWTG-R) registry may also have led to improved survival over time. Improved patient outcomes have also been shown with the use of routine mock codes in pediatric hospitals, audiovisual feedback during resuscitation, and postevent debriefing. Additional resuscitation strategies that may have improved outcomes include earlier recognition and management of at-risk patients, better adherence to resuscitation algorithms, improved coordination between code team members, greater emphasis on quality of resuscitation (e.g., high-quality chest compressions with minimal interruptions), and postresuscitation care (e.g., multidisciplinary care).
Risk Factors
The cardiac diseases associated with sudden cardiac death can be divided into previously recognized or unrecognized diseases ( Table 31.1 ). Children with repaired congenital heart disease (CHD) constitute the largest group among the patients with previously recognized heart disease. The group with previously unrecognized heart disease is more challenging because cardiac arrest may be the presenting sign of the abnormality. Children with unrecognized heart disease may have underlying structural heart disease such as hypertrophic cardiomyopathy, coronary anomalies, or arrhythmogenic right ventricular dysplasia, or nonstructural heart disease with conduction system abnormalities such as long QT syndrome, Wolff-Parkinson-White syndrome, primary ventricular tachycardia/fibrillation, or commotio cordis. Commotio cordis is an underappreciated syndrome in which low-energy impact to the chest wall leads to ventricular fibrillation because of impact during the vulnerable period just before the peak of the T wave. Many of the entities in the group with previously unrecognized heart disease have an underlying genetic predisposition.
WITH PREVIOUSLY RECOGNIZED HEART DISEASE | WITH PREVIOUSLY UNRECOGNIZED HEART DISEASE | ||
---|---|---|---|
Congenital | Acquired | Structural Heart Disease | No Structural Heart Disease |
Tetralogy of Fallot | Kawasaki syndrome | Hypertrophic cardiomyopathy | Long QT syndrome |
Hypoplastic left heart syndrome | Dilated cardiomyopathy | Congenital coronary artery abnormalities | Wolff-Parkinson-White syndrome |
Transposition of the great arteries | Myocarditis | Arrhythmogenic right ventricular dysplasia | Primary ventricular tachycardia and ventricular fibrillation |
Aortic stenosis | Myocarditis | Commotio cordis | |
Single-ventricle palliative procedures—Fontan, Glenn, hemi-Fontan | Primary pulmonary hypertension | ||
Marfan syndrome | |||
Eisenmenger syndrome | |||
Congenital (or postoperative) heart block |
Retrospective analysis of pediatric IHCAs entered into the administrative Kids’ Inpatient Database (KID) revealed survival after cardiac arrest was higher among pediatric surgical cardiac patients (52%) than among pediatric medical cardiac patients (43%); however, children with single-ventricle disease had a lower survival rate (35%) than did children with other forms of cardiovascular disease (45%). Variables associated with an increased risk of cardiac arrest on multivariate analysis in the KID inpatient database included age less than 1 year, heart failure, myocarditis, single-ventricle physiology, and coronary artery pathology, whereas patients undergoing cardiac surgery had decreased risk of cardiac arrest. Single-ventricle patients demonstrated a fivefold increased odds of arrest over CHD patients with a biventricular circulation, and they were the only group with increased odds of death after CPR, even after adjusting for age, hospital characteristics, and comorbidities.
More recent data on the epidemiology of cardiac arrest in cardiac ICUs were explored by the PC 4 registry, which analyzed 15,908 medical and surgical encounters from 23 North American centers. Medical encounters had a 50% higher rate of cardiac arrest compared with surgical encounters. On multivariable analysis, prematurity, neonatal age, any Society of Thoracic Surgeons preoperative risk factor, and Society of Thoracic Surgeons–European Association for Cardio-Thoracic Surgery mortality category 4 or 5 had the strongest association with surgical encounter cardiac arrest. In medical encounters, independent cardiac arrest risk factors were acute heart failure, prematurity, lactic acidosis greater than 3 mmol/dL, and invasive ventilation 1 hour after admission. Return of spontaneous circulation occurred in 64.5%, and ECPR was used in 27.2% of cardiac arrest events. Unadjusted survival was 53.2% in encounters with cardiac arrest versus 98.2% without cardiac arrest. Medical encounters had lower survival after cardiac arrest (37.7%) versus surgical encounters (62.5%), with the Norwood surgical population noted to have less than half the survival after cardiac arrest (35.6%) compared with all others.
The AHA’s GWTG-R multicenter registry found significantly improved hospital survival after resuscitation from an IHCA in children who have undergone cardiac surgery compared with children without cardiovascular disease. Among the cardiac patients the survival to hospital discharge in those with surgical cardiac disease (37%) was significantly higher compared with patients with medical cardiac disease (28%) (adjusted odds ratio, 1.8; 95% confidence interval [CI], 1.3-2.5; P < .001), and non–cardiac disease (23%) (adjusted odds ratio, 1.8; 95% CI, 1.4-2.4; P < .001). Additionally, a report from GWTG-R found that children with cardiac disease were more likely to survive to hospital discharge compared with children without cardiac disease when ECPR was used.
The most recent multi-institutional data from the Society of Thoracic Surgeons Congenital Heart Surgery Database (STS-CHSD) suggests a 2.6% cardiac arrest rate for postoperative patients and a mortality rate of 49.4% among those having cardiac arrest. This publication and previous literature have identified younger age, prematurity, genetic syndromes, preoperative comorbid conditions, and increased surgical complexity as risk factors for cardiac arrest in patients following cardiac surgery. As hospitals increasingly develop dedicated cardiac ICUs to optimize the care of this complex patient population, practitioners must understand the unique cardiac arrest incidence and outcome data of this population to improve quality of care and clinical outcomes. Specific causes of arrest, the availability of specialized invasive monitoring, and access to interventions and management subsequent to the arrest may explain the differences in survival in these three large retrospective studies.
Overview of Guidelines for Cardiopulmonary Resuscitation
When managing a cardiac arrest patient in the cardiac ICU, it is important for all team members to have a shared mental model of the goals of the resuscitation. The most common approach to defining the goals of the resuscitation would be to use regionally published, evidence-based resuscitation guidelines such as the AHA, European Resuscitation Council, or Australian and New Zealand Committee on Resuscitation guidelines for basic life support (BLS) and pediatric advanced life support (PALS). These guidelines should be used as the default, and then the team can consider alterations to personalize the resuscitation based on specific aspects of the size of the individual patient, disease population, or situation not yet covered in the standard guidelines, such as how to approach arresting ICU patients with an open chest, presence of hemodynamic monitoring information from central venous lines, arterial lines, near-infrared spectroscopy (NIRS), and special considerations based on a patient’s anatomy (e.g., single ventricle).
Until 2015 the AHA updated their CPR guidelines every 5 years for both BLS and PALS; it has now shifted to a rolling approach (i.e., with the intent to perform updated reviews as new literature is published). In 2010 the guidelines most notably changed the traditional sequence of basic CPR from A-B-C (airway, breathing, circulation) to C-A-B (circulation, airway, breathing) to decrease time to chest compressions and reduce decreased perfusion time. Differences in time to return of spontaneous circulation (ROSC) and clinical outcomes with this change are yet to be determined; however, time to chest compressions and to starting ventilation strategies have both been shown to be decreased with the C-A-B sequence.
New to the 2015 AHA pediatric BLS guidelines is the idea of compression-only CPR—particularly in the setting of a witnessed cardiac arrest that is presumably nonasphyxial in nature or when a provider is unable or unwilling to perform assisted breaths and most likely in the out-of-hospital setting. When a witnessed sudden cardiac death event occurs, there should be high suspicion for unrecognized heart disease as the cause (see Table 31.1 ). In these patients, compression-only CPR has been shown to have better outcomes than no CPR at all. However, in all cases, compression-only CPR has been shown to have worse survival compared with conventional CPR for children. The AHA pediatric BLS guidelines recommend conventional CPR for all pediatric cardiac arrests (regardless of cause) when bystanders are able and willing. In 2017 the first updated, rolling review of this topic reviewed the most recent evidence supporting this but again stated if rescuers were not willing or able to provide mouth to mouth, to perform compression-only CPR rather than not performing CPR.
The parameter goals for the AHA 2015 pediatric BLS guidelines can be seen in Table 31.2 . The AHA 2015 updated PALS CPR algorithm can be seen Fig. 31.1 .

A focus of the guidelines is to standardize and optimize CPR quality, specifically adequate compression rate and depth. For all ages the recommended compression rate is 100 to 120 per minute (same as for adults). For infants and children (up to onset of puberty), adequate depth of compressions is at least one-third of anterior-posterior chest diameter (typically 4 to 5 cm in children) with full recoil of the chest between compressions. The compressor should rotate every 2 minutes to limit provider fatigue, and interruptions in chest compressions should be minimized in an attempt to maximize perfusion to vital organs and to maintain coronary perfusion pressure (CoPP).
Recent literature has shown that early placement of an advanced airway during CPR does not improve survival or neurologic outcome. Because of the lack of definitive evidence, the current PALS algorithm suggests one consider advanced airway placement (either a laryngeal mask airway or endotracheal intubation) during resuscitation if an experienced health care provider is present, with focus on minimizing interruptions in chest compressions. When an advanced airway is present, a ventilation rate of one breath approximately every 6 seconds (10 breaths/min) is recommended with adequate, but not excessive, chest rise. Hyperventilation and overdistention of the lungs should be avoided because this could impede venous return to the thoracic cavity. Presence of an advanced airway also allows continuous end-tidal carbon dioxide (ETCO 2 ) monitoring, which can assist in guiding quality chest compressions, though specific values to guide therapy have not been established in children. This is discussed in detail later in the chapter. If no advanced airway is present, effective bag-mask ventilation should be performed at a rate of 2 breaths per 15 chest compressions (or 2 breaths for every 30 compressions in the setting of one health care provider) with focus on adequate, but not excessive, chest rise.
Drug Therapy
Vasopressors
Theoretically, vasopressors are indicated in cardiac arrest to cause systemic vasoconstriction (increase blood pressure) to increase coronary blood flow, providing perfusion to the myocardium, and increase myocardial contractility of the stunned myocardium. Despite the fact that epinephrine is the key drug used during the management of pediatric cardiac arrest, and the first drug listed in every PALS algorithm, there is little human evidence that vasopressors are effective in helping to achieve return of circulation or impact clinical outcomes. One adult study did show that epinephrine administration was associated with increased return of circulation in OHCAs, but no such pediatric data are available. Currently the only recommended vasopressor in pediatric cardiac arrest is epinephrine (0.01 mg/kg intraosseous [IO]/intravenously [IV] or 0.1 mg/kg per endotracheal tube [ETT]) every 3 to 5 minutes.
Antiarrhythmic Therapy
When a patient experiences pulseless ventricular tachycardia or ventricular fibrillation, CPR and defibrillation (2 to 4 J/kg) should be initiated per the PALS algorithm (see Fig. 31.1 ). If defibrillation is not successful in converting to a perfusing rhythm, amiodarone (5 mg/kg IO/IV bolus) or lidocaine (1 mg/kg IO/IV bolus, 2 mg/kg per ETT) may be given in addition to repeat defibrillation attempts. In a recent systematic review, lidocaine and amiodarone were found to be comparable, so either is acceptable in this setting.
Sodium Bicarbonate
In the past the use of sodium bicarbonate during CPR had been a common practice in an attempt to buffer the metabolic acidosis associated with poor perfusion because acidemia impairs myocardial contraction and cardiac output. In recent years its use has become controversial because some studies have associated its use with worsened outcomes. The administration of sodium bicarbonate during CPR is now recommended only during resuscitation for cardiac arrest specifically related to severe metabolic acidosis, hyperkalemia, and tricyclic antidepressant overdose.
Calcium
Routine calcium administration is not recommended during resuscitation unless there is documented hypocalcemia, calcium channel blocker overdose, hypermagnesemia, or hyperkalemia. Calcium chloride or calcium gluconate may be used; however, calcium chloride should be given through central venous access when possible (i.e., to avoid infiltrates). However, in a true cardiac arrest situation, risk-benefit analysis would suggest the risk of infiltrate should not outweigh rapid administration if clinically indicated.
For a full description of initial dosing of drugs commonly used in the treatment of low cardiac output syndrome in an attempt to avoid a cardiac arrest and during treatment of a cardiac arrest see the Pharmacology: Typical Doses and Indications table in the AHA scientific statement on CPR in infants and children with cardiovascular disease.
Special Considerations for Cardiopulmonary Resuscitation in Patients With Underlying Cardiac Disease
Single-Ventricle Resuscitation
The resuscitation of the child with single-ventricle anatomy is dependent on anatomic factors and cardiopulmonary interaction. Resuscitation profiles for the structurally normal heart and the single-ventricle palliated states are delineated in Table 31.3 , in which the pathway of each circulation and the impact of chest compressions, chest recoil, and positive-pressure ventilation are shown.
Physiology | Circulation Description | Circulation of Blood | Chest Compressions | Chest Recoil | Positive Pressure Ventilation |
---|---|---|---|---|---|
Structurally normal heart | Two-ventricle series circulation without heart disease | Systemic veins—lungs— Pulmonary veins—body |
| Increases the transthoracic gradient from the systemic veins to the RA increasing RV filling | Decreases the transthoracic gradient from the systemic veins to the RA decreasing RV filling |
Stage I Norwood or shunted physiology | Single-ventricle parallel circulation with shunt-dependent PBF | Systemic veins—single V — Lungs (via shunt) or body | Single-ventricle compression results in PBF (shunt ± PVR) and SBF (SVR) | Increases filling to the preload-dependent single ventricle | Decreases filling to the preload-dependent single ventricle |
Bidirectional Glenn and hemi-Fontan | Single-ventricle parallel circulation with PBF dependent on multiple arteriolar vascular beds | IVC—single V—body/brain— SVC—lungs—pulmonary veins—body | Single-ventricle compression results in SBF |
| Decreases filling to the single ventricle by impeding SVC flow and IVC filling |
Fontan | Single-ventricle series circulation | Systemic veins—lungs— Pulmonary veins—body | Single-ventricle compression results in SBF |
| Decreases filling to the single ventricle by impeding both SVC and IVC flow |
Risk Factors for Cardiac Arrest and Death
Anatomic, hemodynamic, and other comorbid factors that contribute to hemodynamic compromise and early death in the infant with single-ventricle physiology include (1) the presence of hypoplastic left heart syndrome (HLHS), pulmonary atresia with intact ventricular septum with right ventricle–dependent coronary circulation, and total anomalous pulmonary venous connection; (2) reduced cardiac function and hemodynamically significant atrioventricular and/or semilunar valve regurgitation; and (3) prematurity and the presence of a genetic disorder or syndrome. Neonates with a univentricular physiology are at higher risk of cardiopulmonary arrest secondary to (1) increased cardiac work from volume overload, (2) unbalanced systemic and pulmonary blood flow (i.e., elevated pulmonary-to-systemic blood flow ratio [Q p :Q s ]), and (3) shunt thrombosis. The risk of cardiac arrest remains high until the superior cavopulmonary anastomosis is completed. The incidence of IHCA in patients with HLHS after stage I Norwood palliation is higher after modified Blalock-Taussig shunt (MBTS) than after right ventricle to pulmonary artery shunt (RVPAS). However, there is no difference in hospital mortality based on shunt type. The Single Ventricle Reconstruction Trial revealed that one-third of infants after stage I Norwood for HLHS died or underwent heart transplantation at 12-month follow-up, with a nontrivial incidence of cardiac arrest and mortality occurring between 30 days after the stage I palliation and the time of the superior cavopulmonary anastomosis (CPA) procedure (interstage period). Thus the interstage period is considered a prearrest state that warrants active monitoring and intervention to improve survival.
Resuscitation in the Patient With Patent Ductus Arteriosus or Shunted Single-Ventricle Physiology
The prearrest state usually includes some combination of lactic acidosis, intestinal hypoperfusion and feeding intolerance, renal insufficiency, or ST wave changes on ECG indicative of coronary ischemia. Q p :Q s is increased in patients with large shunt size relative to body weight. In the neonate with significantly elevated Q p :Q s , increases in systemic vascular resistance (SVR) may result in rapid deterioration with extreme pulmonary overcirculation and shock (“SVR crisis”). Hemodynamic compromise in a shunt-dependent physiology can typically be reversed with inotropic support, preload modification, mechanical ventilation and sedation/analgesia, manipulation of SVR and pulmonary vascular resistance (PVR), and anticoagulation if shunt obstruction is suspected.
Recognition and Management of Shunt Obstruction.
Decreased systemic arterial oxygen saturation after the Stage I Norwood palliation may result from insufficient pulmonary blood flow, intrapulmonary shunting and pulmonary venous desaturation, and/or diminished mixed venous saturation (SvO 2 ). Insufficient pulmonary blood flow may result from mechanical obstruction of the shunt, elevated PVR, inadequate shunt perfusion pressure, or from pulmonary vein stenosis or restrictive atrial communication. Systemic desaturation with normal systemic blood flow and unchanged arteriovenous oxygen saturation difference (initially) is consistent with shunt obstruction. One clinical sign of shunt obstruction in patients who are intubated and mechanically ventilated, and thus have ETCO 2 continuously monitored, is a sudden decline in ETCO 2 with an increase arterial PaCO 2 . The risk of shunt thrombosis is higher in those patients after stage I Norwood palliation with a MBTS than with an RVPAS during the interstage period.
Strategies for prophylactic anticoagulation include heparin therapy in the early postoperative period after shunt placement, followed by enteral aspirin once feeds are initiated. Aspirin may reduce the risk of shunt thrombosis and cardiac arrest during the first year after placement.
Acute shunt obstruction may be treated by (1) oxygen to maximize alveolar oxygenation, (2) bolus dose heparin (50 to 100 U/kg) for rapid anticoagulation, (3) systemic vasoconstricting medications to maximize shunt perfusion pressure (e.g., phenylephrine, norepinephrine, epinephrine), (4) cardiac catheterization or surgical intervention to open shunt, and (5) use of extracorporeal life support (ECLS) to support circulation. Therapies that decrease PVR (e.g., oxygen, hyperventilation, inhaled nitric oxide) will provide little benefit if there is complete shunt occlusion. When shunt obstruction occurs with reduced oxygenation, sedation, and neuromuscular blockade, the placement of an advanced airway and mechanical ventilation at low mean airway pressure will reduce oxygen consumption and may maintain oxygen saturation while the patient is waiting for definitive therapy to open the shunt. If shunt obstruction results in prolonged and severe arterial desaturation, cardiac function will be impaired quickly. In infants with acute shunt obstruction, ECPR may be beneficial if used promptly to support the myocardium during the acute obstruction and/or afterwards during myocardial recovery.
Unique Challenges in Cardiopulmonary Resuscitation in the Patient With Patent Ductus Arteriosus or Shunted Single-Ventricle Physiology.
Cumulative data from the STS-CHSD from 2007 to 2012 revealed a 13% incidence of cardiac arrest in those patients who have undergone stage I Norwood palliation. When the infant with a shunted single-ventricle physiology has cardiac arrest, it is difficult to obtain ROSC. The mortality rate for infants after stage I Norwood palliation who develop cardiac arrest is five times higher than for those infants who do not suffer cardiac arrest. When cardiac arrest develops, providers should begin conventional high-quality CPR.
It is difficult to obtain effective pulmonary blood flow during resuscitation in the infant with shunted single-ventricle physiology because pulmonary blood flow is shunt dependent and is impacted by PVR and aortic diastolic pressure (for MBTS) and SVR (for MBTS and the RVPAS). Compressions generate only one-third of normal blood flow to the heart and brain during CPR in patients with normal cardiac anatomy. In patients with single-ventricle shunted physiology, systemic blood flow during CPR is very likely to be even lower secondary to the loss of some systemic cardiac output to the pulmonary circulation due to the parallel circulation (see Table 31.3 ). In the neonate with shunted physiology in cardiac arrest the systemic blood flow (SBF) often has low oxygen content and in the presence of reduced coronary perfusion will result in coronary ischemia. As a result, the neonate with shunt-dependent single-ventricle physiology who undergoes a cardiac arrest with prolonged CPR is at particularly high risk for significant end-organ injury, including neurologic injury.
Due to the limitations of conventional CPR in the infant with shunt-dependent physiology, it is important to consider other resuscitation measures early in the resuscitation, including use of a pacer for bradycardia (particularly if pacing wires are already available), treatment of arrhythmias, treatment of possible shunt thrombosis, urgent opening of the sternum (in the immediate postoperative period), and early activation of ECLS, if available in the institution. In the infant with shunted single-ventricle physiology, prolonged in-hospital resuscitative efforts that include ECPR may be successful, whereas out-of-hospital resuscitation efforts are typically not successful.
Superior Cavopulmonary Anastomosis and Fontan
In the child with a superior CPA circulation with low cardiac output or respiratory insufficiency or failure, strategies to improve venous return to the superior CPA will increase pulmonary blood flow and improve systemic saturation and systemic oxygen delivery. Ventilatory strategies that promote relative hypoventilation to increase cerebral blood flow and minimize intrathoracic pressure have been shown to be most useful. Although spontaneous breathing is preferable to augment the extrathoracic to intrathoracic pressure gradient and increase pulmonary blood flow and stroke volume in the superior CPA or Fontan physiology, judicious mechanical ventilation is typically well tolerated in circumstances of respiratory insufficiency or failure or low cardiac output. Positive pressure ventilation lowers systemic afterload and wall stress to the systemic ventricle for the child with poor ventricular function or atrioventricular valve regurgitation; however, positive pressure ventilation will decrease pulmonary blood flow and ventricular preload. Modulation of inotropic support, afterload reduction, and positive pressure ventilation is complex and must be individualized for each child in an effort to avoid cardiac arrest. Table 31.4 delineates the effect of respiratory manipulations on circulatory parameters at different stages of palliation of children with univentricular physiology.
Stage | Respiratory Strategy (Alveolar Gas) | SaO 2 | SvO 2 | Q p /Q s | TPG | ΔAVO 2 | VO 2 | Lactate | CBF | rSO 2 C | rSO 2 S |
---|---|---|---|---|---|---|---|---|---|---|---|
0 | Hypocapnic Hyperoxic | ||||||||||
Hypercapnic Hypoxic | ↑ ↓ | ↑ ↓ | ↓↓ | ↓ ↔ | ↑ ↔ | ||||||
1 | Hypocapnic Hyperoxic | ↔ ↑ | ↔ ↑ | ↔ ↔ | |||||||
Hypercapnic Hypoxic | ↔ | ↔↑ | ↔ | ↓ | ↓ | ↓ | ↑ | ↑ | ↓ | ||
2 | Hypocapnic Hyperoxic | ↓ ↑ | ↔ | ↓ | |||||||
Hypercapnic Hypoxic | ↑↑ | ↔ | ↑ | ↓ | ↓ | ↓ | ↑ | ↑ |
Unique Challenges in Cardiopulmonary Resuscitation in Patients With a Superior Cavopulmonary Anastomosis or Fontan.
Only 1% of patients undergoing a Fontan procedure will develop postoperative cardiac arrest, but 40% of those who arrest will not survive. When cardiac arrest develops in patients who have had either of these surgeries, providers should begin conventional high-quality CPR.
There are important physiologic differences between single-ventricle patients with a superior CPA and a Fontan that impact resuscitation (see Table 31.3 ). In these populations, chest compressions create systemic blood flow; however, pulmonary blood flow can be minimal, resulting in reduced oxygenation and preload to the systemic ventricle, creating a vicious cycle that limits cardiac output. Full recoil in these populations is particularly important (i.e., chest recoil can result in blood flow through the superior CPA and lungs, as well as from the inferior vena cava [IVC] into the systemic venous atrium, providing important preload to the single ventricle for the next compression). In contrast, chest recoil in Fontan physiology results in filling of the total cavopulmonary connection from the superior vena cava (SVC) and IVC. During CPR, cardiac output in patients with a superior CPA may be further reduced when there is hemodynamically significant insufficiency of the atrioventricular or semilunar valves. A further concern in patients with a superior CPA is the reduction in cerebral blood flow and risk of neurologic injury during chest compressions secondary to the elevation in SVC and cerebral venous pressure, minimizing the necessary gradient to perfuse the brain.
Standard Versus Open Chest Cardiopulmonary Resuscitation
There are no data to support changing the AHA-recommended CPR technique in children with CHD, although the effectiveness of chest compressions may be compromised if the patient has an open chest. In addition, there are no data to support changing the techniques for cardioversion and defibrillation in children with CHD.
In defibrillators with pads that contain an accelerometer to measure quality of chest compressions, mattress deflection can confound the measurement of chest compression depth. Thus, when possible, the recommendation would be to use anterior-posterior positioning of these pads such that more accurate measurement of chest compression depth can be made between the pads. These pads can be placed over a sternal dressing to measure quality of chest compressions but will not be able to defibrillate. If the child has dextrocardia, defibrillator pads should be placed over the right side of the chest to keep the heart between the defibrillation pads. If the child’s chest is small, placing the pads in the anterior-posterior position will prevent overlap. This can even be done with paddles for very small infants (i.e., turn the infant on his or her side and place paddles on the chest and back to avoid paddles touching each other). For patients with an open sternum or sternal dressing that require defibrillation, paddle and pad positions may need to be modified, or internal paddles can be used under sterile conditions. In the setting of an open sternum and internal paddles, the dose for defibrillation is 10 to 20 J in adults and 0.6 to 0.7 J/kg in children.
Monitoring Effectiveness of Resuscitation During Cardiac Arrest
Cardiac arrest is a no-flow or low-flow (during CPR) state that risks ischemic injury to critical organs if perfusion is not promptly restored. CPR is the providers’ attempt to restore perfusion and prevent ischemic injury. The prevention of ischemic injury to critical organs depends on early recognition of inadequate perfusion, early institution of resuscitation efforts, the effectiveness of the resuscitation efforts, and the ability to restore adequate spontaneous (or extracorporeal) circulation. Patients with serious cardiovascular disease at risk for cardiac arrest are usually monitored in intensive care, where early recognition and intervention for cardiac arrest are possible. After the early recognition and intervention for cardiac arrest, the intensivist needs to be able to monitor the effectiveness of resuscitation so that efforts can be maximized while attempting to achieve ROSC or initiate ECPR. Monitoring the effectiveness of resuscitation efforts is a rapidly developing field that focuses on maximizing the provider’s performance and optimizing the patient’s response.
Maximizing the Provider’s Performance—Human Feedback and Human Factors
There is increasing recognition of the cognitive load involved in managing a cardiac arrest. It is nearly impossible to ensure exquisite CPR while simultaneously following the rhythm-specific PALS algorithm, identifying and treating reversible causes, and activating ECPR. This is particularly true in the cardiac ICU setting, where teams can be large (including nurses, respiratory therapists, pharmacists, intensivists, and surgeons), there are many streams of information relevant to the cardiac arrest (human voices, bedside monitor with multiple physiologic parameters, NIRS monitor, ETCO 2 monitor, defibrillator feedback), and the anatomic and physiologic considerations of the cardiac arrest may be complex. To address this, Hunt et al. developed a model that explicitly distributes the workload of a resuscitation and intentionally cognitively unloads the team leader. Hunt introduced the role of the “quality CPR coach,” who is responsible for coaching the team members performing CPR (i.e., compressions and ventilations) to perform within the AHA guidelines (see Table 31.2 ) or other physiologic goals such as diastolic blood pressure, CoPP, or ETCO 2 defined by the team leader and to periodically update the team leader. The goal is for the team leader to be able to focus on advanced life support (identifying the cardiac rhythm and following the appropriate PALS algorithm and considering and treating possible reversible causes) while another team member can focus on ensuring very high-quality basic life support. Another approach that can decrease the cognitive load of the leader and the team is to optimize and standardize the ergonomics of the room. Potential benefits include (1) optimizing sight lines (i.e., enable compressors to see the arterial line diastolic blood pressure or the depth of compressions reflected on the defibrillator directly in front of them), (2) improved communication, and (3) a shared mental model of how the room should be organized and a decrease in the amount of effort to “figure it out” during an emergency, for example, to enable the team to know ahead of time how to set up the room for ECPR. This entire approach is similar to a pit crew in auto racing or a dance team that creates choreography and practices with each other before important events.
Maximizing the Provider’s Performance—Mechanical Feedback
Monitoring the effectiveness of the provider’s performance involves feedback on the quality of the resuscitation efforts. This “mechanical” feedback can be used by the providers to meet recommendations for CPR quality. The AHA identifies the five components of high-quality CPR as providing chest compressions of adequate rate, ensuring chest compressions of adequate depth, allowing full chest recoil between compressions, minimizing interruptions in chest compressions (i.e., maximize “compression fraction”), and avoiding excessive ventilation. The recommendations from the AHA for metrics of CPR performance are listed in Table 31.2 . Performing high-quality CPR improves the outcome from cardiac arrest.
Providing resuscitation efforts without having a system of ongoing training, practice, feedback, and debriefing often fails to promote and ensure quality CPR. The inclusion of real-time audiovisual feedback about the quality (mechanics) of CPR has been effective in improving most CPR metrics in simulation (manikin) and actual clinical scenarios. Audiovisual feedback about CPR mechanics has been used to improve the quality of resuscitation efforts. Studies that have investigated CPR quality in actual clinical scenarios with and without audiovisual feedback are presented in Table 31.5 . Several of the studies show improvements in chest compression rate, depth, fraction, and leaning after audiovisual feedback is enabled. One shows that these metrics improved without feedback over the same time frame. Most of these studies fail to show any improvement in outcome (ROSC, survival to discharge [STD], or neurologic function) despite the addition of audiovisual feedback and improvement in quality, though they were not designed with adequate power to do so (see Table 31.5 ).
Study | CCR (/min) NFB vs. FB | CCD (mm) NFB vs. FB | CCF (%) NFB vs. FB | CCL (%) NFB vs. FB | ROSC (%) NFB vs. FB | STD (%) NFB vs. FB | GNO (%) NFB vs. FB |
---|---|---|---|---|---|---|---|
Kramer-Johansen et al., 2006 | 121 vs. 109 | 34 vs. 38 | 52 vs. 56 | 0 vs. 0 | 17 vs. 23 | 3 vs. 4 | NR |
N = 358, OH | P .001 | P .001 | P .08 | P .08 | P .3 | P .7 | |
Abella et al., 2007 | 104 vs. 100 | 42 vs. 44 | 77 vs. 80 | NR | 40 vs. 44 | 9 vs. 9 | NR |
N = 156, IH | P .16 | P .47 | P .26 | P .58 | P .97 | ||
Hostler et al., 2011 | 108 vs. 103 | 38 vs. 40 | 64 vs. 66 | 15 vs. 10 | 45 vs. 44 | 12 vs. 11 | 10 vs. 10 |
N = 1586, OH | P .001 | P .005 | P .02 | P .001 | P .96 | P .21 | P .85 |
Bobrow et al., 2013 | 128 vs. 106 | 45 vs. 55 | 66 vs. 84 | Not % | 25 vs. 22 | 9 vs. 14 | 7 vs. 11 |
N = 484, OH | NS | OR 2.7 (1.2-6.4) | OR 2.7 (1.0-6.9) | ||||
Couper et al., 2015 | 121 vs. 114 | 45 vs. 53 | 78 vs. 84 | 18 vs. 14 | 35 vs. 50 | 16 vs. 17 | 16 vs. 14 |
N = 249, IH | P .02 | P .001 | P .002 | P .75 | P .26 | P .28 | P .60 |
Couper et al., 2015 | 126 vs. 116 | 50 vs. 49 | 78 vs. 82 | 14 vs. 12 | 39 vs. 42 | 17 vs. 13 | 15 vs. 11 |
N = 400, IH | P .001 | P .38 | P .003 | P .91 | P .60 | P .83 | P .86 |
Couper et al., 2015 | 120 vs. 116 | 46 vs. 54 | 80 vs. 84 | 14 vs. 12 | 52 vs. 56 | 19 vs. 20 | 18 vs. 18 |
N = 746, IH | P .08 | P .001 | P .001 | P .87 | P .66 | P .73 | P .80 |
Couper et al., 2015 | 122 vs. 115 | 46 vs. 54 | 79 vs. 83 | 15 vs. 12 | 45 vs. 51 | 18 vs. 18 | 17 vs. 16 |
N = 1395, IH | P .005 | P .009 | P .002 | P .98 | P .03 | P .35 | P .85 |
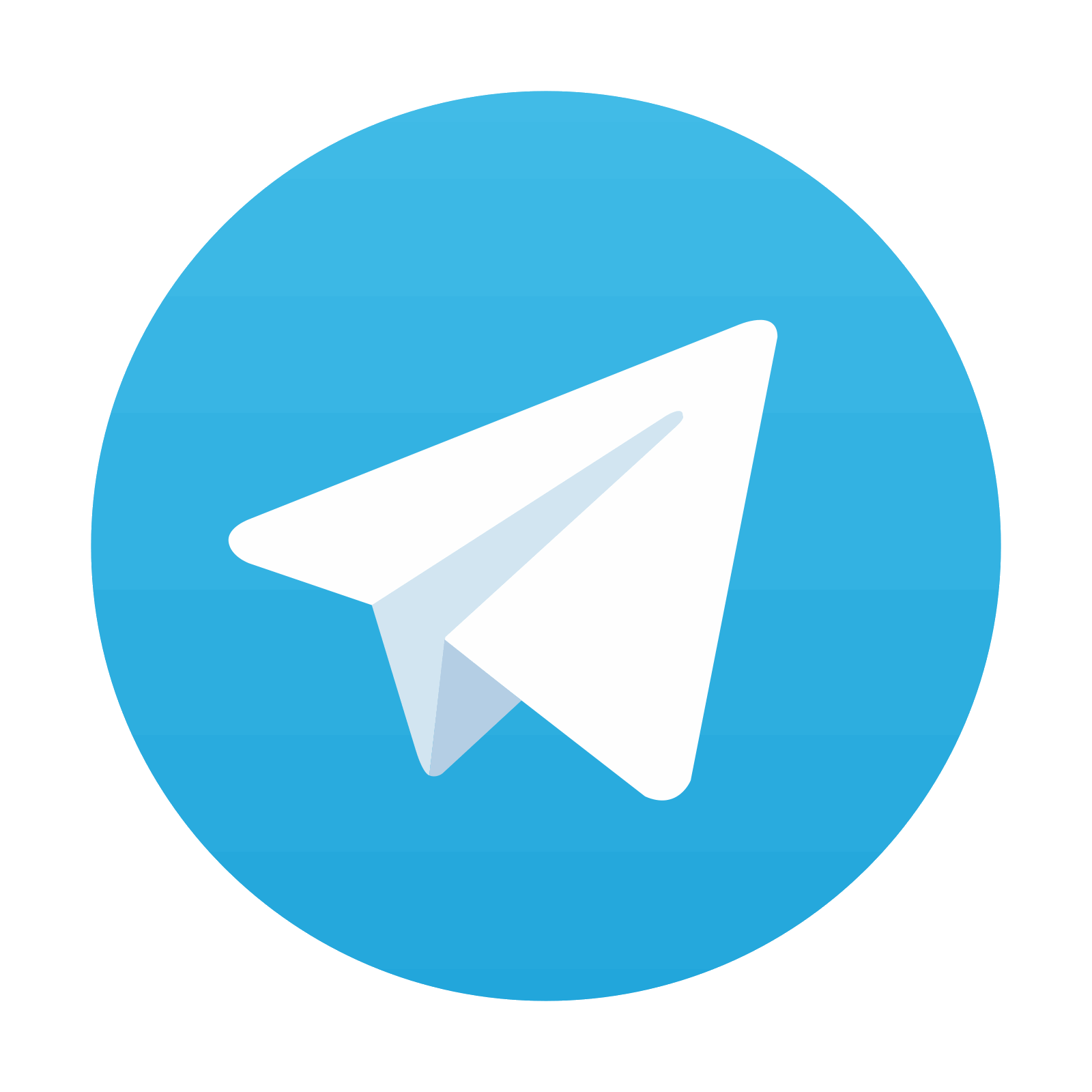
Stay updated, free articles. Join our Telegram channel
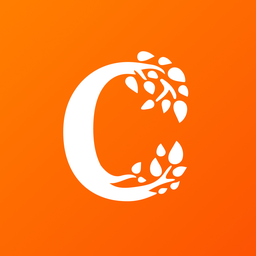
Full access? Get Clinical Tree
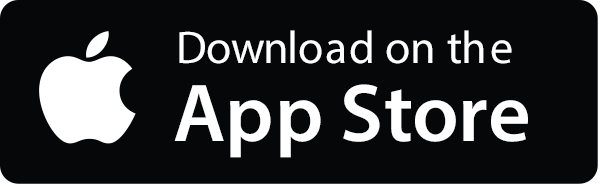
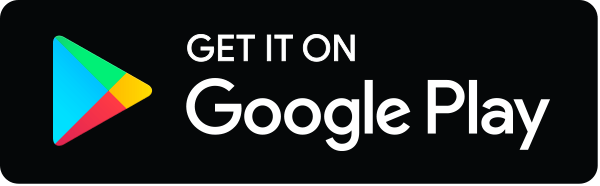
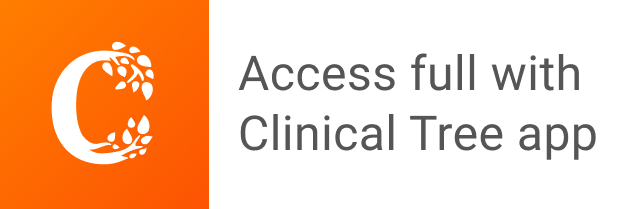