Chapter 3 Cardiopulmonary function testing
ADULTS
ASSESSMENT OF PULMONARY FUNCTION
Introduction
In health the human cardiorespiratory system has enormous reserve capacity to cope with the demands of exercise or illness. We are not normally aware of breathlessness or fatigue as a feature of resting activity. Furthermore, unless we harbour athletic ambitions, we are unlikely to explore the boundaries of our physiological limitations and assure ourselves that spare capacity would be present if it ever became necessary. The measurement of physiological capacity in health is, therefore, a matter of relevance only to the curious or the serious competitor who wishes to improve his performance. In patients with heart or lung disease the erosion of physiological reserve eventually imposes limitations upon the activities of daily life. Under these circumstances the measurement of cardiopulmonary function allows the accurate assessment of disability and of the effect of therapeutic intervention. This chapter examines the scientific basis of clinical measurement and its relevance to physiotherapy.
Lung function
General principles of measurement
Lung function measurements are made to describe the lung for diagnostic purposes and subsequently in monitoring change. Accuracy and consistency are therefore very important and conventions exist for the procedures of measurement and expression of results. In general, a measurement will only be accepted after multiple attempts have been scrutinized and expressed under standard conditions. These are usually body temperature and atmospheric pressure (BTPS). To guarantee accuracy, laboratory practice should include regular physical and biological calibration of the equipment. Standards for good laboratory conduct have been described (British Thoracic Society/Association of Respiratory Technologists and Physiologists 1994). In health there are several factors that influence the magnitude of lung function. These include height, sex and age and to a lesser degree weight and ethnic origin (Anthonisen 1986, Cotes 1993). As a result, assessment of normality can only be made by comparison with reference values. The latter are obtained from the study of large numbers of normal people from the relevant population (European Community for Coal and Steel 1983). Once obtained, results can be expressed as percentage predicted or, more correctly, by comparison with the 95% confidence interval for that value.
Airway function
Production of the spirogram from a maximal forced expiration following a full inspiration is reliable and provides the forced expiratory volume in 1 second (FEV1) and the forced vital capacity (FVC) (Fig. 3.1). The measurement is usually made using a spirometer, which measures volume, or is derived from a flow signal obtained from a pneumotachograph or turbine. Most commonly, the FEV1 and FVC are measured during the same manoeuvre, but a greater vital capacity may be obtained in patients with airway disease if it is performed slowly. Reduction in FEV1 with relative preservation of FVC or vital capacity (VC) is known as an ‘obstructive’ pattern, which indicates and grades airway obstruction: FEV1/FVC <75% is graded as mild, <60% as moderate and <40% as severe impairment (American Thoracic Society 1986). Simultaneous reduction in both FEV1 and FVC with an increase in the FEV1/FVC ratio is called a ‘restrictive’ defect and is usually associated with a reduction in lung volume. Abnormal values are defined as those recognized to be outside the normal range of two standard deviations for sex, height and age. This usually requires a reduction of about 15% from predicted values. Thus simple spirometry can detect and quantify airway obstruction, but gives no indication of the cause.
Measurement of the flow–volume curve is now commonplace and can provide information about the nature of airway obstruction. In this test, the gas flow from a full maximum expiration is plotted against the expired volume as the lung empties (Fig. 3.2). The flow of gas from the lung reaches a peak expiratory flow (PEF) after about 100 milliseconds and then declines linearly as the lung empties. If the measurement is continued into the subsequent full inspiration, a flow–volume ‘loop’ is produced and inspiratory flow rates can be measured. The shape of the expiratory and inspiratory portions are different, since in expiration the active expulsion is assisted by the elastic recoil of the lung while inspiratory flow rates are a reflection of airway calibre and inspiratory muscle strength only. Something of the nature of the airway obstruction can be learnt from consideration of the actual and relative values of PEF, peak inspiratory flow (PIF) and the values of expiratory flow at 50% and 75% vital capacity (MEF50 and MEF75). Simple inspection of the loop is often sufficient to distinguish between rigid upper airway obstruction, intraluminal obstruction in chronic bronchitis and asthma, and the ‘pressure-dependent’ collapse seen in pure emphysema with relative preservation of inspiratory flow rates.
The PEF is one component of the flow–volume manoeuvre that is widely used owing to the availability of simple devices for its measurement. Provided that the patient does not have weak respiratory muscles and has made a maximum effort, the PEF will reflect airway calibre. The absolute values obtained are not particularly helpful unless they are extremely low but the easily repeated measurements can be used to obtain valuable insight into the mechanisms of variable airway obstruction in asthma. There is a normal diurnal variation in airway calibre of about 50 ml/min, which is exaggerated in patients with poorly controlled asthma (Benson 1983). Wider variation will be seen approaching or recovering from an attack and following exposure to trigger factors.
The real value of the PEF lies in its repeatability and its portability. The issue of meters to patients with asthma allows domiciliary and occupational investigation of asthma. PEF also provides an objective measurement for patients to use to monitor their asthma as part of a self-management plan and it can be used during hospital admissions to record the progress and predict the discharge of patients with airway disease. Although this is valuable in asthma where the airway obstruction is variable, it can show no change at all in patients with chronic airflow limitation in spite of a clinical improve ment. In this case the twice-weekly measurement of FEV1 and FVC is more likely to mirror progress than will the slavish recording of the PEF chart (Gibson 1995).
Changes in spirometry are poorly related to clinical improvements after bronchodilator therapy in chronic obstructive pulmonary disease (COPD). O’Donnell et al (1999) have suggested that an increase in inspiratory capacity (a reflection of resting lung hyperinflation) may reflect improvements in exercise endurance capacity and dyspnoea more accurately than FEV1 or FVC measures. Airway responsiveness is a measure of the degree of airway narrowing to specific and non-specific stimuli. Histamine and methacholine are the most widely used non-specific stimuli in challenge tests. Using the tidal breathing method (Juniper et al 1994), doubling concentrations of methacholine (0.03 to 16 mg/ml) are nebulized via a Wright nebulizer. Airway hyperresponsiveness is defined as a >20% fall in FEV1 with a concentration of <8 mg/ml (PC20FEV1 <8 mg/ml).
Most asthmatics have a combination of eosinophilic airway, airway responsiveness and variable airflow obstruction to the extent that many definitions of asthma now include these three features. There is evidence that directing treatment at improving airway responsiveness reduces mild exacerbations of asthma. Elevated exhaled nitric oxide concentration due to increased inducible nitric oxide synthetase (INOS) expression and activity in the bronchial epithelium is a feature of untreated asthma (Kharitonov et al 1994). The relationship between nitric oxide (NO) and asthma severity or response to treatment is unclear (Sont et al 1999).
The physical properties of the lung
For obvious reasons direct measures of lung volume cannot be made. The most familiar method is helium dilution, which involves rebreathing through a closed circuit a mixture of gases containing a known concentration of helium, which is not absorbed into the circula tion. The measurement of the final concentration of helium is used to calculate the gas dilution, or the ‘accessible’ volume, of the lung. An alternative method uses the Boyle’s law principle: gas in the chest is compressed and the change in pressure is used to calculate the volume of gas within the chest. This method requires a large airtight box or plethysmograph. In both the actual volume that is estimated is the FRC, and total lung capacity (TLC) and residual volume (RV) are obtained from an additional spirometric trace. A further method involves the calculation of the total volume of the lung from the dimensions of a chest radiograph. This volume includes the total volume of gas, tissue and blood. Since the techniques do measure different aspects of volume, consistency in sequential measurements is important. In normal lungs the results are very similar, but where there is airway obstruction the values may be disparate. Such disparity can be used to advantage, e.g. in calculating the degree of trapped gas as the difference between the plethysmographic and helium dilution lung volumes.
The chest wall and the respiratory muscles
The respiratory muscles include the diaphragm as the major muscle of inspiration and the intercostal muscles and scalenes. The latter, together with the sternomastoids, are known as the ‘accessory muscles’, but actually have a stabilizing role in tidal breathing. The combination of the respiratory muscles and the bony rib cage is called the ‘chest wall’ and conceptually is considered as the organ which inflates the lungs. Weakness of the respiratory muscles will eventually lead to ventilatory failure which may first become apparent during the night as an exaggeration of the normal nocturnal hypoventilation (Shneerson 1988).
The function of the respiratory muscles is difficult to study directly since the muscles have complex origins and insertions. Furthermore, their product, which is the pressure generated within the thoracic cavity, depends on the coordinated action of many muscles, the individual functions of which may be difficult to distinguish in life. It is possible to make some assessment of both the strength and endurance of the muscles and also to separate the diaphragm from the other muscles. The simple strength that the inspiratory and expiratory muscles can generate as pressure is easy to measure. The maximum inspiratory pressure (Pimax) and expiratory pressure (Pemax) are easy to measure with a manometer or electronic gauge. The normal values of approximately −100 cmH2O and +120 cmH2O (Black & Hyatt 1971) are well in excess of that needed to inflate the lungs (5–10 cmH2O) and therefore provide a sensitive measure of developing muscle weakness. These measurements do have a learning requirement and are not suitable for monitoring of patients with rapidly developing muscle weakness, such as in Guillain–Barré syndrome. Under these circumstances the sequential measurement of the vital capacity is much more reliable, since a failure to maintain it will predict ventilatory failure.
The strength of the diaphragm can be separated from the other muscles by measuring the pressure gradient across it. This is achieved by using balloons attached to pressure transducers to estimate the pressure in the oesophagus and the stomach. The gradient across the diaphragm during a maximum inspiration or sniff is an indirect measure of the strength of the diaphragm. Normal values for sniff pressures have now been published (Uldry & Fitting 1995). If required, a value free of volition can be obtained by electrical stimulation of the phrenic nerve in the neck or even by magnetic stimulation of the cerebral cortex.
Fortunately, measurements of separate diaphragm strength are seldom required in clinical practice. A simple guide to diaphragm function can be obtained by observation of the change in vital capacity with posture. When supine, the vital capacity normally falls by 8–10%, but when diaphragm weakness is present it may fall by more than 30%. The measurement of the supine vital capacity is therefore a good screening test of diaphragm function (Green & Laroche 1990) and the measurement of sniff pressures at the mouth or nose is a reflection of pure diaphragmatic activity.
Gas exchange and oxygen delivery
The requirements of the average cell for oxygen are quite modest and a mitochondrion may need a PO2 of as little as 1 kPa (7.5 mmHg) to function effectively. At sea level the atmospheric PO2 is 20 kPa (150 mmHg) (FiO2 = 0.21) and in the process of delivering oxygen to the cell, there is a loss along this gradient. The first step is the dilution of inspired air with expired air within the alveolus. Each tidal breath VT contains a portion of gas which will remain within the airways and not come into contact with the alveoli. This is known as the ‘dead- space ventilation’ VD and must be achieved before any effective alveolar ventilation VA can take place:
Oxygen carriage and arterial blood gases
The normal atmospheric PO2 is approximately 20 kPa (150 mmHg) falling to 16 kPa (120 mmHg) within the alveolus. The arterial PO2 (PaO2) is usually about 14 kPa (105 mmHg) in a healthy subject. Although we are used to these values, they are only true at sea level and really only have relevance because the partial pressure is easy to measure. What matters to the individual cell is the quantity of oxygen that it receives, not the partial pressure. Oxygen delivery to the tissues depends on other factors, including the amount of haemoglobin, the degree of saturation of haemoglobin with oxygen and the rate at which oxygenated blood is delivered to the tissues. Assuming that the haemoglobin and the cardiac output are normal, then the measurement of oxygen saturation of haemoglobin is more relevant to oxygen delivery than is the PaO2. The PaO2 is related to oxygen saturation in a complex manner determined by the properties of haemoglobin and known as the ‘oxygen dissociation curve’ (Fig. 3.3). This relationship demonstrates that, under most conditions, once PaO2 reaches 8 kPa (60 mmHg), haemoglobin is fully saturated and cannot carry more oxygen. Thus an arterial PO2 above that value is only an insurance measure.
The availability of pulse oximeters has made the non-invasive measurement of oxygen saturation (SpO2) comm-onplace. Pulse oximeters work by transcutaneous examination of the colour spectrum of haemoglobin, which changes with its degree of saturation. These instruments are reasonably accurate over the top range of saturation, but become unreliable below about 50% (Tremper & Barker 1989). The measurement of SpO2 is an extremely valuable tool for monitoring patients’ safety. There are, however, some important aspects of interpretation of its use that may be potentially hazardous. Oximetry provides information about oxygen saturation and this will relate to ventilation only if the inspired oxygen level is normal. Monitoring oxygen saturation will not detect underventilation and a rising PaCO2. In patients who are breathing additional oxygen, a false sense of security can be given by a normal SpO2 even though the PaCO2 is rising. Furthermore, accurate recording of SaO2 requires a good peripheral circulation which may often be compromised in patients who are hypovolaemic.
The assessment of acid–base status requires the measurement of arterial blood gas tensions. The average blood gas analyser measures PO2, PCO2 and pH. It subsequently calculates from the Henderson–Hasselbalch equation the values of bicarbonate, standard bicarbonate and base excess. The appreciation of the acid–base state requires examination of PaCO2 and pH. Abnormalities are usually described in terms of their generation (Fig. 3.4). For example, a respiratory acidosis resulting from underventilation will display a low pH and an elevated PaCO2. If this has been present for any length of time the serum bicarbonate will have become elevated and acid is excreted by the kidneys to compensate. In cases of nocturnal hypoventilation the daytime PaO2 may be normal, but the elevation of the base excess gives a clue to the ventilatory history. If an alkalosis (high pH) is associated with a low PaCO2, then this could be due to voluntary hyperventilation and is termed a ‘respiratory alkalosis’. The build-up of acid products in diabetes or renal failure will result in a low pH and bicarbonate together with a low PaCO2 in an attempt to compensate for a metabolic acidosis. Finally, the loss of acid from the stomach in prolonged vomiting can produce a metabolic alkalosis, which is characterized by high pH, high bicarbonate and normal PaCO2. These sketches of blood gas disturbance are superficial interpretations, but they provide a useful framework for clinical management under most circumstances.
Respiratory failure
The understanding of respiratory failure has changed in recent years with the recognition that it is seldom due to a single malfunction of the respiratory system. For example, the rise in PaCO2 and hyperinflation associated with worsening airway obstruction may adversely affect the respiratory muscles and introduce a chest wall contribution to failure. Conversely, the loss of lung volume associated with muscle weakness may lead to atelectasis and decreased pulmonary compliance, which will in turn put a greater load on the lung. Understanding of the complexities of chronic respiratory failure has helped to improve the outlook for some groups of patients, e.g. those with ventilatory failure due to chest wall disease or obstructive sleep apnoea. In these conditions there are abnormalities of breathing during sleep, which may result in nocturnal hypoventilation or transient apnoea that produce periods of oxygen desaturation which may spill over to the daytime. Recognition of this by oximetry and other more detailed somnography may result in effective treatment by nocturnal nasal intermittent positive pressure ventilation or continuous positive airway pressure (CPAP). By extension these techniques may also have a place in the acute management of selected patients with COPD who have diminished respiratory drive (Wedzicha 1996).
Oxygen prescription
The prescription of oxygen for patients with COPD is well defined for long-term (more than 15 hours/day) use. An indication for long-term oxygen therapy (LTOT) is a PaO2 less than 7.3 kPa, when breathing room air during a period of clinical stability. Clinical stability is defined as the absence of exacerbation of COPD and of peripheral oedema for the last 4 weeks. Ambulatory oxygen is defined as ‘oxygen delivered by equipment that can be carried by most patients during exercise and activities of daily living’ (Ram & Wedzicha 2004). It should be provided to individuals who are on LTOT, need to be mobile and to leave the house. Patients without chronic hypoxaemia and LTOT should be considered for ambulatory oxygen if they have demonstrable desaturation on exercise. It is suggested that the level of desaturation should be at least 4%, to a saturation below 90%, on a standard exercise test while breathing room air. An improvement of at least 10% in distance and/or breathlessness score on repeat testing with supplemental oxygen warrants the prescription of an ambulatory system. Lacasse et al (2005) found that ambulatory oxygen had no effect on the Chronic Respiratory Questionnaire or the 6-minute walk test (6MWT) distance completed after a trial of domiciliary ambulatory oxygen. A second study did, however, report modest improvements in quality of life with domiciliary ambulatory oxygen (Eaton et al 2002). The prescription of short-burst oxygen is less well defined. There is no evidence to support firm recommendation and further research is required to establish its place.
Posture and thoracic surgery
Knowledge of the effect of posture and thoracic surgery on pulmonary function is obviously very important to the physiotherapist. The circumstances of treatment make this knowledge of practical benefit. Lung function measurements are usually made sitting or standing, but the major postural effect occurs due to gravity in the supine position. There is a small fall in VC (8%) and a reduction in FRC while lying down, which results from repositioning of the diaphragm and pooling of blood in the chest. This change can be used to advantage to identify patients with covert diaphragm weakness where the VC may drop by more than 30%. Gravity also produces a change in the distribution of ventilation and perfusion within the lungs. In the supine posture, ventilation and perfusion are preferentially directed to the dependent zones (Kaneko et al 1966). This is important in adults if the lung disease is unilateral, since oxygenation will be better if the good lung is dependent.
Physiotherapists are often involved in the assessment of patients for cardiothoracic surgery and their subsequent management. Some thoracic surgery, such as lung volume reduction surgery (American Thoracic Society 1996
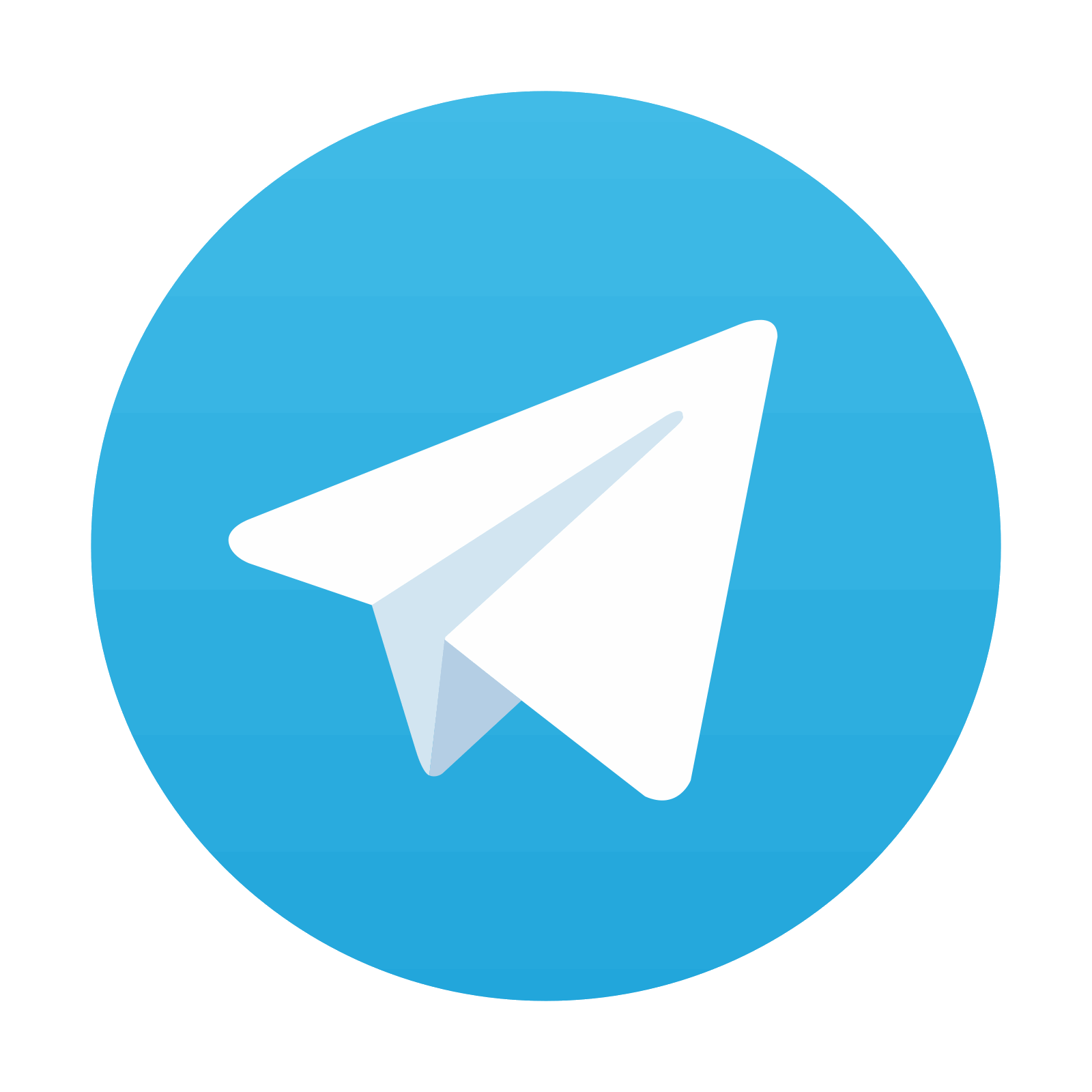
Stay updated, free articles. Join our Telegram channel
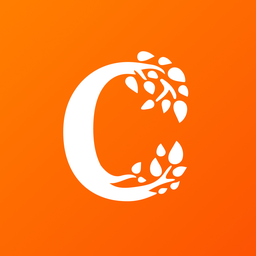
Full access? Get Clinical Tree
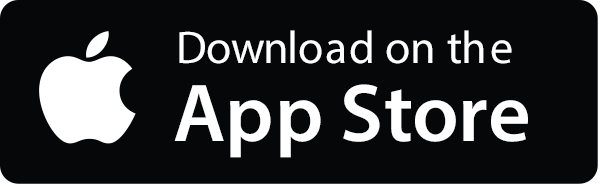
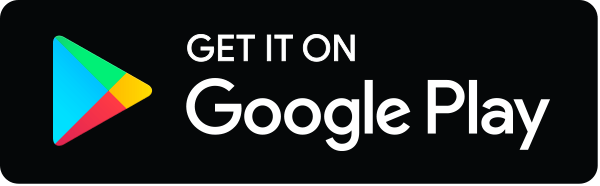