Abstract
Cardiopulmonary bypass in neonates and infants poses significant unique risks that translate into postoperative management challenges. These challenges are best met when the intensivist understands the impact of cardiopulmonary bypass on the various end-organ systems, their individual manifestations, and the tools available in our armamentarium to prevent and treat them.
Key Words
cardiopulmonary bypass, postoperative bleeding, low cardiac output syndrome, renal failure, myocardial protection
Until the advent of hypothermia and the heart lung machine, cardiac surgery was limited to palliative operations performed “outside” the heart. Tetralogy of Fallot was palliated with a subclavian artery to pulmonary artery shunt that was pioneered by Alfred Blalock, Vivien Thomas, and Helen Taussig in 1943. In 1952 F. John Lewis performed an atrial septal defect (ASD) repair by direct suture using hypothermia (28°C) and inflow occlusion. Shortly thereafter in May 1953, John Gibbon performed the first successful open-heart operation using a pump-oxygenator, repairing an ASD in a young girl. Unfortunately, all his subsequent attempts were met uniformly with failure. The following year, C. Walton Lillehei at the University of Minnesota performed the first of 45 successful intracardiac repairs using controlled cross-circulation by using adult volunteers as live cardiopulmonary bypass (CPB) circuits during the repair of congenital intracardiac defects. Although this technique provided the ability to perform intracardiac repairs with significant success, the pump times were 6 to 40 minutes and cardiotomy times 4 to 14 minutes, which highlights the speed with which these repairs were performed. This speed was driven in part by surgeons not truly knowing the “safe period” for bypass and in part by the relative simplicity of their understanding of congenital heart defect anatomy. Further, the risk to both donor and patient seemed unreasonably high, and for these reasons the technique of cross-circulation with volunteer “heart-lung bypass donors” did not gain popularity. In 1954 John Kirklin at the Mayo Clinic modified the existing heart-lung machines and went on to perform the world’s first successful, reproducible series of open-heart operations using a heart-lung machine. The modifications he and his colleagues made to the original pumping and oxygenator system revolutionized cardiovascular surgery, and CPB circuits in use today have evolved into more elegant, technologically superior, and significantly smaller versions of his original model.
Cardiopulmonary Bypass in Neonates and Infants
CPB in neonates and infants has significant differences in comparison to CPB in adolescents and adults. Depending on a wide range of variables, some patient specific (e.g., age, weight, underlying condition being repaired) and some specific to the system (such as oxygenator type, circuit size and prime volume, timing and need for additional blood products, target temperature during CPB, flow rates, use of and type of cardioplegia, use of reduced flow or circulatory arrest), the response to CPB in neonates and infants can contribute in important ways to their postoperative intensive care unit (ICU) course ( Box 41.1 ). Compared with the typical use of CPB in adults, neonatal (and infant) cardiac repairs often require more profound levels of hypothermia (including temperatures <28 ° C), hemodilution (and the need for blood prime), and low perfusion flow rates (including extended periods of low flow or even interrupted [circulatory arrest] flow). Anatomic abnormalities like aortopulmonary collaterals or interrupted aortic arch require alteration of bypass strategies and/or cannulation techniques. Furthermore, the reality is that unlike coronary bypass grafting, which is a procedure performed on the surface of the heart, most congenital heart repairs are truly intracardiac, and this influences cannulation such that bicaval cannulation—often of extremely small caval vessels—is the norm rather than the exception. Significant hemodilution in neonates and small infants is unavoidable due to large circuits and prime volume relative to their intravascular volume. Hemodilution results in lower hematocrits and dilution of clotting factors and plasma proteins, increasing the risk for dilutional coagulopathy. Immature organ systems have an impact as well (i.e., the production of vitamin K–dependent clotting factors by the liver is diminished, increasing the patients’ propensity for coagulopathy and postoperative bleeding).
Smaller circulating blood volume
Potential for systemic inflammatory response related to large circuit relative to patient size
Need for blood products, particularly in the prime
Higher oxygen consumption rate
Reactive pulmonary vascular bed
Presence of intracardiac and extracardiac shunting
Immature organ systems
Altered thermoregulation
Poor tolerance to microemboli
Neonates and infants require much higher flow rates per body surface area to meet metabolic demands. Neonates are often perfused at flow rates up to 200 mL/kg/min. As temperature is reduced, flow rates can be decreased. This has the benefit of producing less blood in a small and complicated surgical field. Furthermore, in some instances patients are cooled to profoundly hypothermic temperatures (e.g., 15°C to 18°C), and the pump is then turned off (deep hypothermic circulatory arrest [DHCA]). This provides the surgeon with the opportunity to remove the cannulas from the patient and to perform a precise repair in an operative field unencumbered by blood, cannulas, or other apparatus related to CPB. Small children, however, have impaired thermoregulation that requires significant attention to temperature monitoring. When CPB is resumed, patients are rewarmed to their normal temperature, but the effects of profound hypothermia on enzyme systems and on the immediate convalescence of the patient cannot be underappreciated.
In general the immature brain tolerates oxygen deprivation better than the mature brain. This supports the clinical observations that infants tolerate longer periods of DHCA better than older children or adult patients. The lungs are also immature at birth, and lung development proceeds up to approximately 8 years of age. At birth the number of alveoli present is approximately one-tenth of what it is in the adult. The lungs of the neonate are quite fragile and have increased potential for pulmonary edema and hypertension. The kidneys of neonates and infants have high vascular resistance with preferential blood flow away from the outer cortex. Sodium reabsorption and excretion, concentrating and diluting mechanisms, and acid-base balance capacity are limited. These characteristics all influence the response of neonates and infants to CPB. Finally, the immune system of the neonate is immature. Complement generation is impaired, and neonatal mononuclear cells are dysfunctional. These differences and many more make CPB in the infant and neonate a specialized endeavor that requires great attention to detail and the ability to adapt to unexpected situations. As more experience and understanding has been gained with regard to the conduct of CPB, the impact that it has on postoperative patients and their outcomes has become increasingly evident.
In contrast to the previous editions of this textbook, this chapter will characterize specific postoperative challenges faced in the ICU following exposure to CPB, the CPB-specific factors that contribute to the development of these challenges, and the treatment options (preoperative, intraoperative, and postoperative) available to mitigate and minimize some of these potentially deleterious effects of CPB.
Basic Cardiopulmonary Bypass Terms, Conditions, and Strategies
To better understand the relationship between CPB and postoperative convalescence, it is helpful to have a basic understanding of how CPB is provided and of some of the common CPB strategies used by the surgical team. For patients to be placed on CPB, they require anticoagulation. This is usually accomplished with heparin and is managed by following indicators during surgery of adequate heparinization. In the past many centers monitored anticoagulation during CPB using the activated clotting time (ACT), but there have been numerous published reports demonstrating the lack of correlation between heparin levels and the ACT. The ACT can be affected by clotting factor abnormalities and is only a reflection of the ability of the blood to clot, not directly of heparin levels. In recent years many centers have shifted to measuring heparin levels (most often by monitoring Anti Xa levels) and antithrombin III (AT III) levels. Neonates in particular may have low AT III levels, and AT III is essential for heparin to work. Consequently, augmentation of the AT III levels (with exogenously administered AT III—either as thrombate or by fresh frozen plasma [FFP]) is now commonly employed before heparin dosing. During CPB the heparin level is monitored at regular intervals, and if AT III levels are adequate, then management of heparin levels can ensure adequate anticoagulation during exposure to the extracorporeal circuitry.
Placing a patient on CPB is similar to the application of veno-arterial (VA) extracorporeal membrane oxygenation (ECMO) in the ICU—there needs to be an arterial “inflow” cannula (most frequently placed directly into the aorta, but in some circumstances it is placed in a femoral artery or even into a side graft sewn to a vessel like the innominate artery) and a venous “return” cannula. (CPB cannot be supported with venovenous cannulation!) In many circumstances for repair of congenital heart defects, it is necessary to enter the cardiac chambers, so venous return needs to recover venous blood from both the superior vena cava and the inferior vena cava directly (two separate cannulas) as opposed to simply one cannula placed into the right atrium. Once the patient has been anticoagulated and cannulated, CPB commences, and flow rates are adjusted to provide adequate tissue perfusion. For neonates and infants these flow rates can be as high as 200 mL/kg/min, so cannulas need to be of sufficient size to support adequate perfusion.
Depending on the size of the patient, as well as on the patient’s starting hemoglobin level, and the amount of solution needed to “prime” the pump, blood products may need to be added to the pump or during CPB. Today it is commonplace for surgical teams to use a “cell saver” that returns any excessive blood loss either to the pump for reinfusion during CPB or into a system where the blood can be processed for reinfusion, thus minimizing the need for additional blood products and blood exposure.
The surgical team can then choose from numerous strategies that can present challenges in the postoperative setting. Unlike ICU-based ECMO, various degrees of cooling might be employed. Many surgical teams will cool patients to a temperature between 25°C and 32°C. This will allow the team to turn down flow rates, if necessary, to improve exposure of the surgical field while decreasing metabolism so that it can tolerate the periods of decreased perfusion. In some situations the team may use more “profound” cooling (employing temperatures as low as 15°C). The team may elect to do this if they are anticipating a more prolonged period of diminished perfusion (such as during aortic arch repair or repair of pulmonary arteries, in which bronchial flow can create interference with the surgeon’s ability to visualize fragile structures and perform delicate suture reconstruction). At these profoundly low temperatures, surgeons may even turn off the CPB flow for periods of time (using DHCA). This can enable them to perform a cardiac repair in a surgical field that is not flooded with blood. They can even remove the cannulas, if their presence hampers surgical repair, because during DHCA there is no CPB, and the cannulas are not needed. Of course, the consequence of DHCA is NO perfusion to vital organs (e.g., kidney, brain), and despite the decreased oxygen need provided by profound hypothermia, there is a risk for subtle to severe levels of organ ischemia and injury depending on the duration of DHCA. Many teams now use DHCA only sparingly and for relatively short duration (exposure times less than 30 minutes between periods of reperfusion).
In many cases the heart chambers need to be opened, and it is helpful for the team to “arrest” the heart so that it is not beating. An open, beating heart is a risk for ejecting air into the circulation. To provide this arrest a cross-clamp is placed on the aorta, and a solution designed to arrest (and protect the heart) is infused proximal to the clamp so that it can enter the coronary arteries. This solution, called cardioplegia, contains a high potassium level, to create diastolic arrest, among other compounds to help decrease the heart’s metabolic need for substrate. Current solutions can help support cardiac arrest for up to 3 to 4 hours (or more)—usually more than enough time for the surgical team to complete the cardiac repair. However, the consequence of cardioplegic arrest is still a period of ischemia (albeit somewhat “protected”) to the heart, from which it will need to recover.
To protect the heart during the period of aortic cross-clamping, ice or iced saline is sometimes placed over the heart. Likewise, ice packs are often placed around the head of infants who are undergoing periods of altered cerebral perfusion (e.g., DHCA). More recently, carbon dioxide (CO 2 ) is often added to the pump during cooling because cooling tends to make blood alkalotic, and this can decrease flow to the brain. Adding CO 2 (called pH-stat strategy) can improve cerebral blood flow (and protection) when periods of DHCA are anticipated.
For patients undergoing repair of the aortic arch, DHCA used to be a common strategy because it was difficult to continue perfusion through an aortic cannula with the aorta wide open. In recent years it has been popular to sew a side graft for arterial perfusion onto the innominate artery. At cold temperatures the flow rate can be decreased to 25 to 50 mL/kg/min, and with a snare on the proximal innominate, flow will not enter the aorta but instead perfuse the cerebral circulation and return through numerous collaterals to the other head vessels and the descending aorta. With clamps or snares selectively placed on the other head vessels and on the descending aorta, it is possible for the surgical team to provide perfusion to the brain and to the organs in the abdomen (e.g., kidney, mesentery) while working on a widely opened aorta. This technique is often called “selective” or “regional” cerebral perfusion and has contributed to less frequent use of complete DHCA.
The effects of all of these strategies, and others, on the neonate or infant are discussed later. Suffice it to say that infants exposed to CPB are generally exposed to varying degrees of hypothermia, some periods of cardiac (and occasionally whole body) ischemia, and dilution of their blood and clotting factors from the pump prime (sometimes requiring transfusion of blood, platelets, and other clotting factors). In the ICU handoff it is commonplace for the surgical team to communicate the total CPB time (although they do not commonly communicate the temperature that the neonate or infant was cooled to). They will generally communicate the aortic cross-clamp time or the DHCA time (but they may communicate the total clamp or DHCA time and not specify how often cardioplegia was reinfused or how often reperfusion was employed during the period of DHCA).
In general the quality of the cardiac repair is probably more relevant than the total CPB time or whether or not the neonate or infant needed additional CPB to revise the repair. However, there is a correlation between length of CPB and convalescent complications or difficulty. (If CPB duration itself were detrimental, then we would likely expect more CPB-related issues from prolonged ECMO; however, even in those circumstances, the outcome is usually related to underlying adequacy of repair or severity of residual disease rather than to duration of exposure to extracorporeal circulation). Rather than focus on actual CPB time, duration of aortic cross-clamping, or whether or not periods of DHCA were used, it may be more helpful to the ICU team to know the following: (1) What is the quality of the repair? Any residual lesions or anatomic problems that might influence convalescence? (2) Were there any problems noted during CPB that might be important to know about in the convalescent period (e.g., arrhythmias, acidosis, poor urine output, difficulty ventilating)? (3) What seems to be most helpful (and most harmful) to this particular patient (such as “the patient respond well to calcium, but not to volume”; or “the patient is being atrially paced for junctional rhythm”)? Although there is certainly an exposure “cost” to patients for CPB, when it is performed well, with adequate perfusion flow rates, attentiveness to cerebral, cardiac, and whole body protection, appropriate circuitry and use of various strategies; and no overt issues related to injury of vessels or other structures from the CPB instrumentation, the influence of CPB on convalescence should be minimal.
Postoperative Low Cardiac Output Syndrome
Low cardiac output syndrome (LCOS) is a well-recognized, common postoperative complication seen following CPB in neonates and infants with a predictable constellation of hemodynamic and physiologic aberrations. Approximately 25% of children experience a decrease in cardiac index of less than 2 L/min/m 2 within 6 to 18 hours after cardiac surgery. Inappropriately managed LCOS is a risk factor for increased morbidity and death and may occasionally be progressive and refractory, requiring a period of “myocardial rest” with extracorporeal life support (ECLS). The approach to managing LCOS should therefore be proactive and preemptive and involve early detection and early institution of therapy to mitigate and reverse its negative effects. Factors associated with the development of myocardial dysfunction include the inflammatory response associated with CPB (from exposure of blood to foreign surface), myocardial ischemia from aortic cross-clamping with inadequate myocardial protection (despite the use of cardioplegia, each heart may be “biologically” different and respond in unpredictable ways to routine application of well-accepted cardioplegia regimens), reperfusion injury (particularly to areas that were hypoperfused preoperatively, such as areas of lung), hypothermia, and ventriculotomies (which can impose actual injury to heart muscle) when performed. Further compromise in cardiac output may occur due to residual or undiagnosed structural lesions or in instances of late presentation with preexisting right ventricular, left ventricular, or biventricular dysfunction. The risk is greatest for neonates and young infants undergoing complex surgical repairs presenting in circulatory collapse, and those infants and children with preexisting ventricular dysfunction.
Certain preoperative, intraoperative, and postoperative strategies have been successfully instituted to prevent or minimize LCOS and involve addressing the metabolic and endocrine response to CPB, preload optimization, inotropic support, judicious afterload reduction and lusitropy, exclusion of structural defects with precise transesophageal echocardiography, mechanical ventilation strategies, and pulmonary vasodilator therapy for pulmonary hypertension.
LCOS is usually reflected by low blood pressure and (when there is normal sinus rhythm) tachycardia—typical hallmarks of “shock.” However, blood pressure can be normal if low cardiac output is associated with increased systemic vascular resistance, and heart rhythm can be deceptive, particularly if there is heart block. Sometimes LCOS can be perpetuated by cardiac rhythm abnormalities. For example, patients in junctional rhythm may lose their “atrial kick,” and this can result in a diminished cardiac output. The ICU team can monitor evidence for tissue perfusion, and in the current era a downward trend on the near-infrared spectroscopy (NIRS) or an upward trend in lactic acidosis (or increasing base deficit) are important signs that cardiac output may not be normal. Typical management strategies are related to improving preload (if appropriate), decreasing afterload (when possible), or improving contractility (with a variety of pharmacologic agents). Making certain that the cardiac rhythm is not abnormal (or in need of pacing) and that the hemoglobin level is adequate to optimize oxygen delivery are also important. In the face of requiring escalating inotropic support, consideration for early placement on ECLS is warranted. It is also imperative to know of (and to investigate for) the presence of any important residual anatomic defects needing revision.
Preoperative and Intraoperative Strategies
Endocrine Response
CPB is associated with tremendous increases in native catecholamines, particularly epinephrine and norepinephrine. This is due partly to surgical stress but also to peripheral vasoconstriction with relative ischemia, lack of pulsatile blood flow, and acidosis. This elevation of catecholamines extends into the postoperative period. It seems that the initial increases in catecholamine levels fall considerably upon reperfusion of the lungs, likely related to the uptake and metabolism of the catecholamines in the lungs. When the lungs are excluded from the circulation (total CPB), there is significant accumulation of norepinephrine. Hypothermia also has the effect of raising the serum catecholamine levels not only by increasing production, but also by decreasing the metabolism and the downregulation of catecholamine receptors. Circulatory arrest also results in increases of catecholamine levels, but it is likely the rewarming, reperfusion phase of circulatory arrest that is most associated with catecholamine surge. Catecholamine levels tend to fall rapidly once normothermia is achieved and bypass is discontinued. It is clear that the level of anesthesia has a great influence on the surge of catecholamines associated with CPB and surgery. The anesthetic management of neonates has been shaped largely based on the findings of Anand and Hickey. High-dose narcotic induction and maintenance can result in reduction of catecholamine levels and reduced postoperative complications. However, there is a rise in serum cortisol levels after induction of anesthesia and surgery. Initiation of CPB causes the cortisol levels to fall secondary to hemodilution. After separation from CPB, levels again begin to rise, and this continues for 24 hours, after which they gradually fall to normal. The effect of ultrafiltration on the levels of glucocorticoids is not known. Preoperative and postoperative steroids have been shown to mitigate the effects of the LCOS after CPB.
It appears that hypothermia and CPB result in a decrease in the level of insulin, as well as decreased peripheral response to the insulin. The net result is an increase in the serum glucose level. The level of insulin increases after reperfusion and rewarming. Glucagon and human growth hormone are released as part of the general stress response. Growth hormone is an anabolic hormone that tends to rise in both adults and children during and after CPB. A few studies show an increase in glucagon level during the CPB period, and there is clearly a gradual rise in glucagon levels after surgery that peaks at approximately 6 hours.
Thyroid hormone levels decrease during the CPB period and into the first several days after surgery. The reasons for this include hemodilution, decrease in thyroxine-binding globulin, and increased glucocorticoid levels. The lowest levels of thyroid hormones seem to be associated with poor outcome. For this reason, triiodothyronine administered intravenously after CPB can be a useful inotropic agent.
Myocardial Protection
Neonatal repair is the preferred approach to many congenital heart defects. As the complexity of and time required for repair increases, so does the need for effective and optimal myocardial protection. There is some laboratory evidence that the immature myocardium has different structural and functional characteristics than the adult myocardium. The immature heart is less compliant, resulting in a very limited range of the Starling curve. The normal neonatal heart is operating at maximal saturation of adrenergic stimulation, and there is an exaggerated negative inotropic response to anesthetic agents and therefore a requirement for greater doses of inotropic agents when they are needed. The immature myocardium relies heavily on glucose as its major substrate and also has a greater reliance on extracellular calcium for calcium-mediated excitation-contraction coupling. It is widely accepted that the immature heart has a greater tolerance to ischemia than the adult or mature heart. However, most of this laboratory data has been obtained with normal hearts. It is unclear what the ischemic tolerance is when there are preexisting conditions such as cyanosis, hypertrophy, or acidosis. Many of these conditions may be present in neonates and infants who require surgical correction of their heart defect and may compromise myocardial protection. Infants and children with chronic cyanosis as a result of inadequate pulmonary blood flow often have increased bronchial collateral flow. This increased blood return to the left heart can result in insufficient myocardial protection by warming the heart and washing out cardioplegia. Hypertrophied ventricles may also have inadequate myocardial protection and suffer from subendocardial ischemia during prolonged cardioplegic arrest periods.
Hypothermia remains the most important factor for successful myocardial protection in infants. Electromechanical arrest, ventricular decompression, and hypothermia all work together to decrease the myocardial oxygen consumption. Topically applied iced saline may be helpful; however, it often interferes with the operative procedure and may result in phrenic nerve palsy. Therefore many groups use topical cooling intermittently. Typically, cardioplegia is delivered through a catheter or needle in the aortic root after the cross-clamp has been applied. Retrograde cardioplegia delivery (via the coronary sinus), however, has received significant attention and is gaining in popularity in neonates. It is particularly useful in neonates requiring prolonged aortic cross-clamp time and in whom delivery of cardioplegia into the aortic root may not be possible (e.g., aortic valve replacement, arterial switch operation). Blood cardioplegia may be superior to crystalloid cardioplegia, especially for longer (>1 hour) myocardial ischemic times.
Typically, cardioplegia solutions should have a calcium concentration that is below the serum concentration. Despite the potential for excessive calcium influx secondary to hyperkalemia-induced membrane depolarization, potassium remains the most widely used cardiac arresting agent in all of cardiac surgery. Magnesium helps to maintain that negative resting membrane potential and also inhibits sarcolemmal calcium influx. The addition of magnesium to blood cardioplegia results in significantly improved functional recovery. Magnesium enrichment of hypocalcemic cardioplegic solutions can result in near complete functional recovery, but even high-dose magnesium supplementation cannot reverse dysfunction in severely stressed hearts that receive normocalcemic cardioplegia. Typically an induction dose of 30 mL/kg cardioplegia solution at 4°C is delivered. Although there is no conclusive evidence either to support or refute the practice, many surgeons will use multiple doses of cardioplegia at approximately 20- to 30-minute intervals during the ischemic period. This practice may be helpful when there are significant bronchial collaterals, or in operations performed using mild or moderate hypothermia, both of which may result in premature warming of the heart. Single-dose or reduced frequency del Nido cardioplegia, which has a higher concentration of magnesium and lidocaine, has been gaining acceptance in many pediatric cardiac surgery programs. The reduced need to halt the flow of the surgical repair helps reduce the cross-clamp time while maintaining excellent myocardial protection.
In selected operations on the right side of the heart in which the septa are intact, that is, tricuspid valve repair or pulmonary valve repair or replacement (or when aortic cross clamping with administration of cardioplegia may not be feasible, such as when there is significant scarring around or thinning of the aorta), a technique referred to as “empty beating” may be employed, in which case little myocardial ischemia occurs and a cross-clamp is not employed. This is a reasonable technique as long as there is no communication between the right and left sides of the heart through which air can pass and result in air embolus to the systemic circulation. Mild hypothermia with fibrillatory arrest can be used in operations on the right side of the heart, especially when a significant intracardiac communication exists, such as an ASD. This technique is not recommended when there is significant aortic valve regurgitation because severe left ventricular distention will occur.
Intramyocardial air has also been suggested as a contributing factor for myocardial dysfunction after pediatric cardiac surgery. In 4% of 350 consecutive pediatric patients undergoing repair of congenital cardiac defects, intramyocardial air was detected in the immediate postbypass period using intraoperative echocardiography, despite aggressive “deairing” maneuvers before removing the aortic cross-clamp. Hemodynamic instability was apparent in some of these patients, but in many cases the intramyocardial air produced no clinical signs. None of the patients had clinical evidence of air embolism to other organs (e.g., brain). Echocardiographic imaging demonstrated increased echogenic areas localized to the right ventricular free wall and along the inferior portion of the intraventricular septum, suggesting the distribution of air was localized to the area supplied by the right coronary artery. The right coronary artery is a likely source for embolization of retained left ventricular air because of the location of the ostium of the right coronary artery on the anterior aspect of the aorta. Therefore residual left ventricular air is more likely to enter the right coronary artery and result in right ventricular ischemia and dysfunction, except in patients undergoing an arterial switch procedure for d-transposition in which the left coronary artery and left ventricle may be involved. (Following an arterial switch operation, both coronary arteries are anterior, and thus the risk of air embolism to either the right or left coronary distribution is increased.) Deairing is performed with the aortic cross-clamp left in place, and the cardioplegia needle is used to vent air from the left ventricle. Therapy for myocardial air embolism is directed at increasing perfusion pressure to propel air through the arterioles and capillary bed. Dramatic hemodynamic and echocardiographic improvements have been demonstrated in patients with intramyocardial air after the administration of phenylephrine or reperfusing the heart with high pump flow rates and high perfusion pressures on CPB. Some groups “blow” carbon dioxide (CO 2 ) over the operative field to limit the risk of significant air embolus and reduce the risk of neurologic events. (Carbon dioxide is heavier than air, so it displaces air from the pericardial sac during surgery. Carbon dioxide also dissipates from the circulation more readily than air, so it is less likely to cause an air embolus.)
Other adverse effects of CPB include increased capillary permeability and increased total body water (TBW), which often results in tissue edema and multiple organ dysfunction. Intraoperative techniques that have been developed for reversing tissue edema and hemodilution after CPB include conventional ultrafiltration (CUF) during CPB and modified ultrafiltration (MUF), which is performed in the immediate post-CPB period and removes excess water from the patient, as well as providing a method for salvaging blood from the circuit. MUF has been shown to modulate the inflammatory response to CPB by removing inflammatory mediators, including interleukin (IL-6, IL-8), and tumor necrosis factor. A prospective randomized trial of MUF showed improved hemodynamics with a reduction in TBW and decreased need for blood transfusion when compared with nonfiltered controls. MUF has been shown to improve left ventricular systolic function after CPB, resulting in increased systolic blood pressure and cardiac index.
Postoperative Strategies
Postoperative strategies that may be used to manage patients at risk for or in a state of low cardiac output include the use of hemodynamic monitoring, enabling a timely and accurate assessment of cardiovascular function and tissue oxygenation; optimization of ventricular loading conditions; the judicious use of inotropic agents; afterload reduction and lusitropy; an appreciation of and the use of positive pressure ventilation for circulatory support; and in some circumstances mechanical circulatory support. All interventions and strategies should culminate in improving the relationship between oxygen supply and demand, ensuring adequate tissue oxygenation.
NIRS has now become a standard monitoring tool in postoperative ICU assessment and provides a reasonable measure of tissue oxygen delivery and its response to resuscitation. Arterial lactate levels and myocardial oxygen consumption trends are also useful for monitoring hemodynamic optimization.
Maximizing oxygen delivery preload optimization is achieved by correcting the hemoglobin level with packed red blood cells transfusions; positive inotropic support is improved with epinephrine, dobutamine, or dopamine and lusitropy; and afterload reduction is achieved with phosphodiesterase inhibitors such as milrinone. In patients with the vasodilatory form of LCOS, vasopressin and norepinephrine can be judiciously used to normalize afterload. Pulmonary hypertension, right ventricular dysfunction, and cyanosis can be treated with inhaled nitric oxide (iNO) and hyperventilation to reduce arterial CO 2 . Postoperative steroids have been shown to reduce the inflammatory vasodilatory shock seen in LCOS. Calcium infusions have recently received some attention in the management of LCOS in children undergoing CPB. Neonates undergoing cardiac surgery for various univentricular heart staging strategies receive the maximum hemodynamic benefit from calcium, likely due to the fact that the immature myocardium with structurally underdeveloped sarcoplasmic reticulum is most dependent on extracellular calcium levels. Occasionally, however, despite all of these measures persistent lactic acidosis and poor tissue perfusion necessitate ECMO or mechanical support. Early institution of mechanical support before end-organ failure has set in has been shown to improve outcomes in some neonates undergoing the Norwood procedure.
Postoperative Acute Kidney Injury and Fluid Management
Children undergoing CPB tend to develop severe tissue edema from a capillary leak syndrome secondary to the inflammatory response seen during CPB. This increased capillary permeability leads to tissue and subsequent organ dysfunction, particularly myocardial and pulmonary edema. Acute kidney injury, which is common postoperatively and carries substantial morbidity and mortality, complicates postoperative fluid management. Strategies to reduce TBW focus on reducing hemodilution intraoperatively and removing excess volume with diuresis or renal replacement therapy postoperatively.
Intraoperatively, hemodilution may be addressed prebypass, during CPB, and in the postbypass period. Prebypass strategies include circuit miniaturization and the use of colloid or blood prime as opposed to crystalloid. The use of blood versus asanguineous prime is often debated; however, recent evidence suggests that asanguineous prime followed by transfusion as needed may decrease the inflammatory response associated with CPB when compared with blood prime. There is some suggestion in recent studies that circuit miniaturization may obviate the need for MUF and lead to decreased transfusions while on CPB. This, however, is in the setting of substantial evidence that MUF leads to decreased perioperative blood transfusions. Continuous removal of fluid in the venoarterial direction while on CPB is referred to as CUF, as opposed to MUF, which involves a short period of ultrafiltration in the arteriovenous direction immediately after separating from bypass. CUF can be an effective technique; Thompson et al. noted that when amounts of fluid removal are standardized, CUF and MUF resulted in equivalent outcomes measures such as hematocrit, ventricular function, and postoperative transfusions. However, CUF is complicated by the inability to remove large amounts of fluid without having to administer replacement fluid to maintain reservoir levels, whereas MUF has the advantage of filtering the entire circuit and delivering blood filtered to a desired hematocrit back to the patient, resulting in greater hemoconcentration. MUF, with or without preceding CUF while on CPB, has been shown in several studies to be superior to CUF alone with regard to increasing hematocrit, decreased inotrope usage, decreased chest tube output and blood transfusion, and decreased duration of mechanical ventilation. There is some discrepancy in the literature as to the superiority of MUF over CUF; however, outcomes may be affected by technique, circuit arrangements, and the heterogeneity of the studied populations. Flow rates require careful monitoring during MUF, however, because aggressive ultrafiltration can lead to increased runoff from the aorta, compromising cerebral blood flow.
The postoperative mainstay of fluid balance in the ICU remains diuresis; however, standard loop diuretic therapy is usually limited in the first 48 hours due to a blunted response due to a diuretic-refractory oliguric phase. Neonates undergoing complex congenital cardiac surgery commonly develop some mild to moderate acute kidney injury and demonstrate a positive fluid balance in the first 24 to 48 hours with an elevated pressor and inotrope requirement. There is increasing evidence that early peritoneal dialysis (PD), traditionally reserved for patients in renal failure, is a safe and effective way to manage fluid balance. Renal failure is common after pediatric cardiac surgery, and renal failure requiring PD is associated with a high mortality. However, early application of PD by identifying patients at high risk for renal failure per the RIFLE (Risk, Injury, Failure, Loss, End Stage) criteria leads to more net negative fluid balance and decreased mortality, and usage has therefore been expanded in some centers to include those at high risk of renal failure or prophylactically in those with signs of fluid overload. PD was recently compared to diuresis in a randomized trial and found to be superior in reducing fluid overload, ventilator duration, and inotrope requirements. Serious complications of PD catheter placement include hydrothorax, peritonitis, and bowel perforation ; however, these are uncommon, and catheter placement may be accomplished intraoperatively in a transdiaphragmatic fashion, which may be safer than transabdominal placement at a later time. Instillation of dialysate into the abdomen has been shown to be well tolerated, with minimal to no effect on cardiac or pulmonary dynamics.
PD has been shown to reduce the inotrope and pressor need and maintain an even or slightly negative fluid balance during this critical first 24 to 48 hours postoperative period. However, as with any intervention during this critical phase of instability, PD has been shown to be associated with increased morbidity in neonates with hypoplastic left heart syndrome undergoing the Norwood procedure. Acute fluid shifts in some situations may result in worsening hemodynamic instability and outcomes if not carefully monitored.
Hemostasis and Cardiopulmonary Bypass
Bleeding is the Achilles’ heel of complex neonatal congenital cardiac surgery repairs. CPB exerts intended and unintended effects on the enzymatic and formed elements of the coagulation and inflammatory systems. Compared with adults, children are at special risk for known, and unknown, differences in their hemostatic systems. CPB leads to a whole body inflammatory response involving the formed blood elements, coagulation, complement, and kallikrein/kinin systems. Hemodilution is directly related to the volume of crystalloid and colloid required to prime the circuit and the patient’s own blood volume and can dilute blood components by as much as 40%. Although children have relatively larger circulating blood volumes when compared with adults (e.g., preterm infants have an estimated blood volume of 90 to 100 mL/kg compared to 70 to 80 mL/kg in an adult), their low absolute blood volume accentuates their dilutional coagulopathy. Further, their small blood volume creates practical limitations on the use of certain blood conservation strategies such as autologous donation and cell saver. A profound thrombotic reaction involving both the extrinsic and intrinsic coagulation pathways is initiated by continuous exposure of heparinized blood to the perfusion circuit and to the wound during cardiac surgery. Despite large doses of heparin during CPB, heparin does not block thrombin generation but partially inhibits thrombin after it is produced. Thrombin is continuously generated, and a consumptive coagulopathy is initiated. If thrombin formation could be completely inhibited during CPB, the consumption of coagulation proteins and platelets could largely be prevented.
The coagulopathy induced by CPB affects children more profoundly than adults. Neonates are particularly affected, as a result of their unique cardiac lesions, immature hematopoietic and coagulation systems, and small blood volumes. After birth, erythropoiesis profoundly decreases, accompanied by the cessation of fetal hemoglobin production. This physiologic anemia results in hemoglobin nadirs of 9 to 11 g/dL at 8 to 12 weeks of age. Neonates have inherent deficiencies in vitamin K–dependent factors II, VII, IX, and X, which may be corrected by administering vitamin K at birth. Deficiencies in contact factors XI, XII, prekallikrein, and high-molecular-weight kininogen are also present at birth. Hepatic immaturity leading to decreased factor synthesis and accelerated clearance of factors through higher metabolic rates also play a role. Levels of coagulation inhibitors are also low. Protein C, protein S, heparin cofactor II, and AT III levels are 40% to 60% of adult values, slowly achieving adult levels by 180 days. Platelet aggregation may be impaired, and fibrinogen exists in a dysfunctional fetal isoform. Notably fibrinogen level, platelet counts, and levels of factors V, VIII, von Willebrand factor, and XIII are normal at birth. In spite of the preceding, prothrombin time and thrombin clotting time are normal within a few days of birth. Thromboelastography has shown that neonates and infants actually develop faster and stronger clots than adults.
Following surgery, bleeding that persists after the patient is brought to the ICU can be a major contributor to morbidity. The surgical team can likely comment on the possibility that bleeding is mechanical and might require reexploration. Bleeding that exceeds a rate of 10 mg/kg/h is significant. Factor replacement therapy (platelets, fresh frozen plasma, cryoprecipitate, and occasionally recombinant factors IX or VII) has utility, and a thromboelastogram can be helpful in directing therapy. Most important to the management of these patients is close communication between the surgical and ICU teams. It is critical to replace blood loss in the immediate postoperative period, usually milliliter for milliliter to maintain hemodynamics and hemoglobin levels.
Pulmonary Dysfunction
Pulmonary injury can manifest in several ways because the lungs have both a parenchymal and a vascular component. Parenchymal effects of CPB are reflected by alterations in pulmonary compliance most commonly related to an increase in lung water. The impact of this on the patient is a diminished ability of the lungs to perform their function in gas exchange, which may result in a requirement for increased ventilatory support. Endothelial cell dysfunction is exacerbated by CPB, cardioplegic arrest, and hypothermia. The vascular effects are manifested by changes in pulmonary vascular resistance, which in turn affects function of the right ventricle. The lungs are in a unique position in the circulation and may be vulnerable to different mechanisms of injury.
There are multiple specialized cells within the lung, including many cells of inflammation. The lung is an important source and target of the inflammatory response to CPB. Part of the pulmonary derangement that occurs is related to the inflammatory response from CPB. This is manifest as decreased functional residual capacity, compliance, and gas exchange, as well as increased pulmonary vascular resistance and pulmonary artery pressure. Post-CPB acute respiratory distress syndrome can occasionally impair oxygenation and ventilation to a severe degree, eventually requiring temporary ECMO support.
Inflammation is not the only factor that produces impairment of pulmonary function from CPB. When patients are placed on bypass, the lungs have a sudden and significant decrease in antegrade flow via the pulmonary artery. During “total” bypass the lungs receive only “nutrient” flow from their bronchial supply. This relative ischemia of the lungs, in addition to the inflammatory effect of CPB, may result in significant clinical pulmonary dysfunction. It seems that low-flow CPB produces worse pulmonary injury than circulatory arrest, suggesting that the interaction between the inflammatory and ischemic components is complex. Both the inflammatory and ischemic factors produce damage to the pulmonary endothelium, and this leads to increases in post-CPB pulmonary vascular resistance and pulmonary artery pressure, both of which can have significant implications in neonates and small infants, especially after certain types of procedures, such as the Norwood procedure for hypoplastic left heart syndrome.
A number of suggestions have been made for limiting this pulmonary dysfunction. Some intriguing work with Perflubron (liquid ventilation) infused into the lungs before bypass demonstrate that the antiinflammatory and oxygen-carrying capabilities of this compound can have a dramatic effect on postbypass pulmonary parenchymal and vascular function. The use of steroids may lessen the inflammatory response to CPB. Steroids given before exposure to CPB reduce lung water accumulation, improve post-CPB pulmonary compliance, and limit post-CPB pulmonary hypertension. MUF after CPB seems to immediately improve pulmonary function compared with function in patients who do not undergo ultrafiltration. All of this suggests that some significant advances can be made with respect to the pulmonary response to CPB.
Following CPB, patients are at particular risk for life-threatening pulmonary hypertensive crisis (PHC). iNO has been shown to reduce pulmonary hypertension and improve postoperative outcomes in children with pulmonary hypertension undergoing congenital heart surgery with CPB. At low doses iNO has been shown to improve ventilation/perfusion matching, decrease the intrapulmonary shunt fraction, and often increase the systemic arterial saturation. Further, a randomized controlled trial using iNO in the postoperative period after surgery for congenital heart disease demonstrated decreased time to meet criteria for extubation and decreased incidence of PHC. Empiric NO infused into the CPB circuit has also been shown to improve postoperative outcomes.
In patients with significant lung dysfunction following exposure to CPB, increased ventilator requirements can create a form of “pulmonary tamponade” with decreased filling of the heart due to the increased peak ventilator pressures. These patients may benefit from having the surgical team reopen the sternum (if it is closed) to provide less impairment of hemodynamics from temporarily increased ventilation requirements.
Neurologic Injury
In the patient with congenital heart disease, neurologic injury continues to play a significant role in quality of life over the patient’s entire life span. Although historically there were clear causes for severe neurologic injury, they have since been better understood and significantly reduced from the routine treatment of our patient population.
Profound hypothermia with DHCA was historically associated with choreoathetosis, seizures, and other forms of immediately recognizable neurologic dysfunction. With advances in management strategies for hypothermic CPB and more sophisticated use of DHCA (including acid/base blood gas management strategies—particularly for high-risk groups such as those with prominent aortopulmonary collaterals, reduced exposure duration to altered flow and reperfusion techniques, and use of higher hemoglobin during CPB) prominent clinically evident neurologic injury following CPB has become uncommon. Part of the problem in developing strategies to improve the impact of neurologic injury is that it is difficult to separate patient- and disease-related risk factors (over which we have little control) from risk factors related to controllable CPB management strategies.
The actual incidence of neurologic injury after infant cardiac repair, 10% to 25% of patients in some series, highlights the importance of attention to detail in factors affecting the infant brain. This risk of injury can be viewed in three components: (1) preexisting risk associated with various congenital heart lesions and with each individual child’s own unique brain substrate, (2) injury induced by CPB and the various CPB strategies that can be employed by the surgical team, and (3) injury sustained during the “vulnerable” period after exposure to CPB when the patient is in the ICU. When neurologic injury is manifest in a patient after CPB, it is often impossible to determine which of these elements played the most prominent role.
The risk for abnormal neurologic development, even without exposure to CPB or repair of the lesion, is significant and ranges from 2% to 10% for a variety of congenital heart lesions. This may relate to abnormal cerebral perfusion patterns or to actual associated structural anomalies within the brain. Certain cardiac defects associated with syndromes (i.e., atrioventricular septal defects and trisomy 21) have an even higher incidence of abnormal brain development irrespective of whether the patient is exposed to CPB. The natural history of neurologic development without cardiac surgery is not known and is unlikely ever to be known for several serious and life-threatening congenital cardiac lesions because it is rare to leave these lesions unrepaired or for these children to survive without surgery long enough to provide an adequate “control” group. Nevertheless, there is literature to show that cerebral flow patterns are extremely abnormal in infants with congenital heart disease before they are placed on CPB.
Finally, the condition of infants at the time of presentation greatly affects their neurologic outcome. Perhaps the greatest impact on the reduction of neurologic injury in the preoperative period comes from prenatal diagnosis and subsequent delivery of the child in a center equipped to handle the fragile infant with severe heart disease. Nonetheless, careful attention to detail with regard to adequate systemic perfusion, cerebral perfusion pressures, avoidance of hypoxia and perhaps just as importantly hyperoxia, as well as glucose levels and temperature control, are all critically important. Some groups now recommend routine magnetic resonance imaging spectroscopy in neonates scheduled to undergo repair under CPB, especially in infants born preterm, to look for preexisting neurologic injury or substrate for injury such as small intraparenchymal bleeds, immature myelination, or other structural abnormalities. Although the utility of this strategy is yet to be determined, infants with significant presurgical abnormalities can be delayed from cardiac repair, or the increased risk can be communicated to the parents and appreciated by the care team.
The physiology of CPB produces many “alterations” that might relate to neurologic injury. During CPB, microembolic events commonly occur and can contribute to end-organ injury. Alteration in cerebral blood flow can be demonstrated by middle cerebral artery transcranial Doppler, retinal angiography, echocardiography, and infrared spectroscopy, although monitoring with any of these methods has not been clearly shown to be clinically beneficial. Direct evidence of air embolism to the brain can possibly be suggested by visualization of air in the coronary vessels, electrocardiographic changes of ST-segment elevation, and blanching of the skin of the head. Neurologic events have been correlated with the presence of focal dilations of the microvasculature or very small aneurysms in terminal arterioles and capillaries within the cerebral circulation. The use of membrane oxygenators, arterial filters, and adequate heparinization (ACT >400 seconds) for CPB decreases the number of microemboli and may reduce the incidence of embolic events during CPB. Yet despite these methods, air embolism remains an important factor in postoperative neurologic dysfunction.
During pediatric CPB the frequency in which the left side of the circulation is exposed to air increases the likelihood of systemic air embolization. In a report of perioperative neurologic effects in neonates undergoing the arterial switch operation for transposition of the great arteries, the presence of a VSD was associated with an increased incidence of postoperative seizure activity. Although this could be related to longer periods of DHCA (in a historical series when DHCA was used more frequently), the data could also suggest a higher incidence of air embolism as a cause for neurologic dysfunction. When recognized, cerebral air embolism can be treated by attempts to reduce gas bubble size by reestablishing hypothermic CPB or through the use of hyperbaric oxygen therapy in the early postoperative period. Major cerebral air embolism has also been treated with retrograde cerebral perfusion in adult patients, although experience with this modality in children is limited. Both hypothermia and hyperbaric therapy reduce the size of gaseous microbubbles and allow them to pass through the arterial and capillary beds, resulting in reduced ischemia.
Hypothermic Injury to the Brain
Early experience with deep hypothermia suggested that using extremely low temperatures (esophageal temperatures of less than 10°C) resulted in a dramatic increase in neurologic and pulmonary injury. Neurologic sequelae, especially choreoathetosis, were commonly reported. Neuropathologic examination of the brain of animals undergoing profound levels of hypothermia revealed microvascular lesions compatible with the no-reflow phenomenon. Similar histologic brain lesions were observed in children who died after cardiac surgery using circulatory arrest. These early reports diminished the enthusiasm for profound levels of hypothermia, and most institutions “increased” target hypothermic temperatures for DHCA to between 18°C and 20°C.
More recent, and probably more accurate, information suggests that some of these early fears are unfounded. Profound hypothermia does not seem to create cerebral injury and in fact may correlate with improved cerebral protection during periods of DHCA. However, risk for injury may be affected by the type of blood gas management strategy used during DHCA (pH-stat versus alpha-stat), the duration of the time spent cooling, the duration of the period of DHCA, or the presence of significant aortopulmonary collaterals.
The lower limit at which hypothermia causes significant end-organ damage is not known. Current clinical practices suggest, however, that temperatures of 15°C and below are probably no worse than temperatures of 18°C to 20°C. It seems clear that at hypothermic temperatures (<22°C) autoregulation of cerebral blood flow is lost and decreases in a linear fashion with decreases in mean arterial pressure.
It is now well recognized that cerebral blood flow decreases in a linear relationship to decreasing temperature, whereas cerebral metabolism is reduced exponentially as temperature is decreased ( Fig. 41.1 ). This provides most of the cerebral protection afforded to the brain by cooling because cerebral metabolic needs for oxygen are significantly lowered and cerebral blood flow becomes “luxurious.” Nevertheless, cerebral metabolism persists, even at very low temperatures, and the brain is therefore susceptible to ischemic injury despite hypothermia if cerebral blood flow is eliminated (e.g., DHCA) for prolonged periods of time. It is possible to predict the amount of cerebral blood flow required to support cerebral metabolic needs at decreasing temperatures, and this is the rationale behind continuous low-perfusion CPB ( Table 41.1 ).
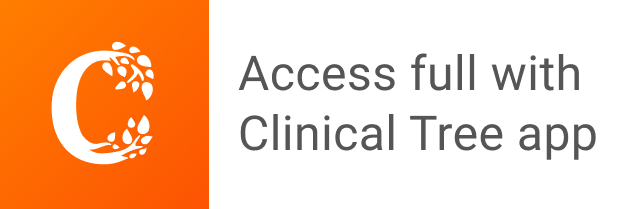