Fig. 27.1
Pathways for the synthesis of long-chain omega-6 and omega-3 polyunsaturated fatty acids. Leukotriene B (LTB); prostaglandin E (PGE); thromboxane A (TXA), prostacyclin (PGI)
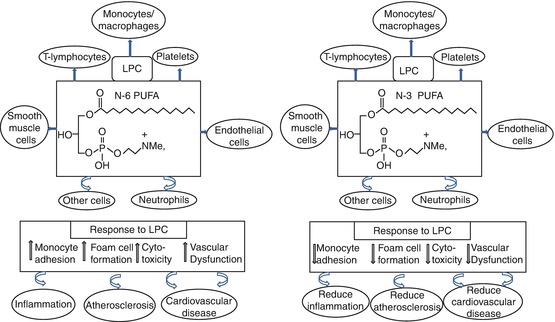
Fig. 27.2
Potential atheroprotective mechanisms of omega (n)-3 polyunsaturated fatty acid (PUFA)-enriched lysophosphatidylcholine (LPC)
27.2.1 n-3 PUFA and Cardiovascular Disease
The scientific inquiry into the health benefits of n-3 PUFA originated from the Bang and Dyerberg’s observation of the Greenland Inuit [25]. They observed that the Greenland Inuit had lower concentrations of plasma TG, LDL and total cholesterol (TC) as compared to the Danish control; these differences were ascribed to the high-fish diet of the Inuit. n-3 PUFAs such as EPA and DHA occur predominantly in fish oil, and an inverse relationship between fish consumption and incidence of CVD has been reported in a meta-analysis of 200,575 subjects [26]; other clinical studies have also corroborated these findings [27,28]. Furthermore, it has been reported that Americans exposed to a high-fish diet had a significantly lower risk of coronary heart disease [29]. Dietary supplementation of n-3 PUFA (>2 g/day) has been shown to alleviate symptoms of dyslipidaemia, improve endothelial function and resolve inflammation associated with the development of CVD [30–32]. There are also evidence supporting the anti-inflammatory, antithrombotic, anti-atherogenic and antiarrhythmic properties of n-3 PUFA [33]. The North American intake of n-3 PUFA is approximately 130–150 g/day [33,34]. With the documented health benefits of n-3 PUFA, the consumption of two fatty fish servings per week for the prevention of CVD was recommended by the American Heart Association [35]; this is estimated to provide EPA and DHA of approximately 450–500 mg/day [35,36]. There is no universally recognized recommendation for the consumption of n-3 PUFA due to dietary variations in different countries. However, most countries and organizations have made different recommendations based on the recognized health benefits of n-3 PUFA. Some of these recommendations accounted for gender, age and health status; however, the common theme among the different recommendations is the n-6 to n-3 PUFA ratio of approximately 5:1 (Table 27.1). The health benefits of n-3 PUFA depends on its availability in the body and its accretion to body tissues; hence, the omega-3 index which is the percentage of the erythrocyte highly unsaturated n-3 PUFA (EPA + DHA) can be considered as a possible risk factor of cardiovascular irregularities [37]. An omega-3 index of 4 % represents low cardioprotective effects, while an index of 8 % signifies relatively high cardioprotection [38]. The premise for this was that the fatty acid composition of the blood can be used as surrogate for the fatty acid composition of the cardiac muscle [39].
Table 27.1
Omega-3 polyunsaturated fatty acid international intake recommendation
Source | Date | n-6:n-3 ratio | Other specific recommendations (%en=% of daily energy intake) |
---|---|---|---|
National Nutrition Council of Norway | 1989 | None | 0.5 % en n-3 LCPUFA (1–2 g/day) |
NATO Workshop on n-3/n-6 | 1989 | None | 0.8 g/day EPA/DHA (0.27 %en) |
Scientific Review Committee of Canada | 1990 | 5:1–6:1 | n-3 PUFA at least 0.5 %en |
British Nutrition Foundation Task Force | 1992 | 6:1 | EPA 0.2–0.5 %en: DHA 0.5 %en |
FAO/WHO Expert Committee on Fats and Oils in Human Nutrition | 1994 | 5:1–10:1 | Consider pre-formed DHA in pregnancy |
UK Committee on Medical Aspects of Food Policy (COMA) | 1994 | None | Fish twice/week, one of which should be oil, minimum intake EPA/DHA 200 mg/day |
Ad Hoc Expert Workshop | 2000 | None | EPA + DHA 0.3 %en:0.65 g/day minimum |
France: AFFSA, CNERNA and CNRS | 2001 | 5:1 | 500 mg n-3 LCPUFA/day: DHA 120 mg minimum |
US National Academy of Science/Institute of Medicine | 2002 | None | 130–260 mg EPA + DHA/day |
American Heart Association | 2002 | None | If no CHD, eat (oily) fish twice/week; if CHD consume 1,000 mg n-3 LCPUFA/day; if high triglycerides, take 2–4 g per day, under medical supervision |
UK Scientific Advisory Committee on Nutrition (SACN) | 2004 | None | Fish twice/week, one should be oily, min intake EPA/DHA 450 mg/day |
ISSFAL | 2004 | None | 500 mg n-3 lcPUFA/day |
Australia and New Zealand Government Recommendations | 2005 | None | n-3 LCPUFA men 160 mg/day; women 90 mg/day |
Superior Health Council of Belgium | 2006 | None | A minimum of 0.3en% EPA + DHA for adults |
Health Council of the Netherlands | 2006 | None | To achieve the dietary reference intake of 450 mg of n-3 PUFA from fish a day, it is necessary to eat two portions of fish a week, at least one of them being oily fish (such as salmon, herring or mackerel) |
27.3 Cardioprotective Mechanisms of n-3 PUFA
Several mechanisms have been proposed by which n-3 PUFA can prevent CVD; the three main mechanisms are (a) regulation of lipid and lipoprotein metabolism, (b) alteration of membrane dynamics and production of bioactive lipids and (c) reduction of inflammation. The following sections focus on the involvement of n-3 PUFA in the regulation of these pathways.
27.3.1 Cardioprotective Effects of n-3 PUFA by Regulating Lipid and Lipoprotein Metabolism
Alterations in lipid metabolism underlie the pathology of diseases such as CVD and diabetes mellitus; thus, the regulation of lipid metabolism is crucial for maintaining physiological function. The following subsections highlight the importance of n-3 PUFA in regulating lipid and lipoprotein metabolism and the associated mechanisms.
27.3.1.1 n-3 PUFA and Serum Lipoprotein Metabolism
The transport of dietary fats from the intestine to the liver and movement of cholesterol to peripheral tissues in mammals are facilitated by lipoproteins [40]. Lipoprotein particle is composed of lipids and apolipoproteins (apo) responsible for its structural integrity [41]. Lipoproteins are categorized according to their particle density and size; the least dense lipoproteins are the chylomicrons followed by very-low-density lipoprotein (VLDL), intermediate-density lipoprotein (IDL), LDL and HDL [41]. The densities of these lipoproteins are defined by the relative concentration of lipids to proteins. The major types of lipoproteins and their properties are given in Table 27.2. During lipid metabolism, ingested dietary fats are transported from the intestine in form of chylomicrons. The TG in the circulating chylomicrons is metabolized by lipoprotein lipase (LPL), transforming them into chylomicron remnant, which is cleared by the hepatic LDL receptor and the LDL receptor-related protein; the released fatty acids are transported to the adipose tissue where they form TG and are stored [43]. The liver synthesizes cholesterol and fatty acids which are packaged and transported in VLDL. Similar to chylomicron metabolism, VLDL also undergoes hydrolysis by LPL to produce free fatty acids which are once again transported to the adipose tissue for storage as TG. VLDL loses its lipids and transforms to IDL which can be cleared by the liver or further metabolized by LPL resulting in a loss of apo-E to produce LDL. LDL contains apo-B 100 and is the main transporter of plasma cholesterol and can be cleared by LDL receptor on the membrane of the liver. LDL has been said to carry the “bad cholesterol,” an excess of which could be highly atherogenic. Conversely, HDL is produced both in the liver and intestine from precursor apoA-1. Lipid poor apoA-1 acquires lipid by the action of ATP-binding cassette transporter (ABCA1) to form nascent HDL particle [44]. HDL is further lapidated by the action of ABCG1 from lipid acquired from TG-rich lipoproteins. Lecithin cholesterol acyltransferase (LCAT) esterifies the cholesterol in the HDL, thereby forming mature HDL [45]. HDL is involved in reverse cholesterol transport which is responsible for carrying cholesterol from peripheral tissues to the liver for excretion; this makes HDL functionally an atheroprotective. Figure 27.3 shows a schematic representation of the lipoprotein metabolic pathway.
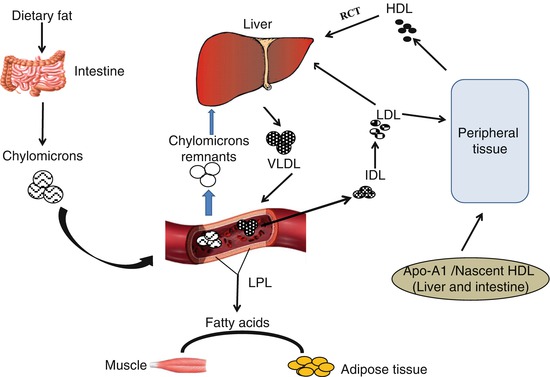
Table 27.2
Properties and functions of lipoprotein classes
Lipoproteins | Density (g/mL) | Apoproteins | Composition (%) | Lipid delivery method | Function | |||
---|---|---|---|---|---|---|---|---|
Protein | TG | Chol | Phosp | |||||
Chylomicron | <0.95 | B-48, C, E | 2 | 90 | 5 | 3 | Lipoprotein lipase hydrolysis | Transport of dietary TG from intestine to hepatic and extrahepatic tissues |
VLDL | 0.95–1.006 | B-100, C, E | 6 | 60 | 20 | 14 | Lipoprotein lipase hydrolysis | Transport of TG from liver to extrahepatic tissues and precursor of IDL |
IDL | 1.006–1.019 | B-100, E | 18 | 20 | 40 | 22 | Receptor-mediated hepatic endocytosis and conversion to LDL | Precursor of LDL |
LDL | 1.019–1.063 | B-100 | 21 | 7 | 50 | 22 | Receptor-mediated endocytosis by the liver and extrahepatic tissues | Primarily transports cholesterol from the liver to extrahepatic tissues |
HDL | 1.063–1.210 | A | 44 | 5 | 25 | 26 | Transfers cholesterol to IDL and LDL | Responsible for reverse cholesterol transport from extrahepatic tissues to the liver |
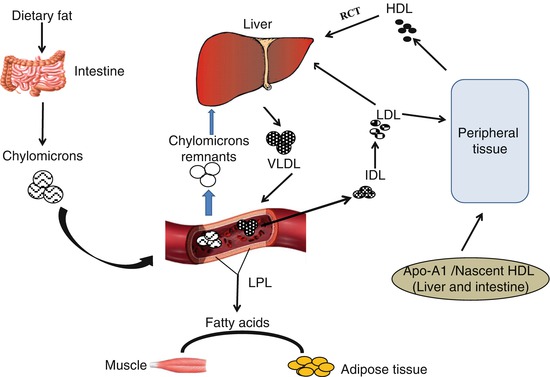
Fig. 27.3
Lipoprotein metabolic pathway. VLDL very-low-density lipoprotein, IDL intermediate-density lipoprotein, LDL low-density lipoprotein, HDL high-density lipoprotein, LPL lipoprotein lipase, Apo-A1 apolipoprotein A1, RCT reverse cholesterol transport
TG is transported in circulation by lipoproteins, primarily VLDL and chylomicrons; the concentration of plasma TG affects the concentration of plasma lipoproteins. TG is associated with atherogenic lipoproteins such as VLDL and LDL; n-3 PUFA reduces TG concentration chiefly by reducing hepatic VLDL production [46]. This also leads to an increased conversion of VLDL to LDL [47], which explains the increase in LDL cholesterol concentration upon supplementation with n-3 PUFA [46]. Individuals supplemented with n-3 PUFA have been shown to have an increase in LDL cholesterol concentrations which is a risk factor of CVD [31,48]; however, the increase in LDL is towards a less atherogenic large buoyant LDL particle. The effects of n-3 PUFA on lipoprotein concentrations have been controversial. The current consensus is that n-3 PUFA improves the quality of lipoproteins profile to a less atherogenic subclass without affecting their concentrations [49]. Studies have shown that small dense LDL is capable of infiltrating the arterial wall and prone to oxidation, making them more atherogenic compared to the large buoyant LDL particles [50]. n-3 PUFA has been shown to increase the concentration of less atherogenic large LDL particles [51]; this is facilitated by a decrease in TG concentration which causes an increase in LDL particle size as a result of an increase in hepatic VLDL clearance [52].
High circulation TC level is also a risk factor for the development of CVD [53]. n-3 PUFA regulates TC concentration by downregulating the expression of sterol regulatory element-binding protein (SREBP), thereby suppressing the expression of 3-hydroxy-3-methyl-glutaryl CoA (HMG-CoA) reductase, the key enzyme of cholesterol biosynthesis [54]. Liver X receptor (LXR) also prevents cellular cholesterol accumulation by upregulating the expression of 7-α-hydroxylase, cytochrome P450 [CYP7A], an enzyme involved in bile synthesis, thus converting excess cholesterol into bile [55]. High-density lipoprotein (HDL) is involved in reverse cholesterol transport and has been colloquially referred to as the carrier of good cholesterol. There are reports showing an increase in HDL cholesterol concentration upon supplementation with n-3 PUFA [56]. HDL exerts its cardioprotective effect mainly through reverse cholesterol transport, which is the removal of excess cholesterol from extrahepatic tissues to the liver for excretion. The functionality of HDL depends on its ability to remove cholesterol which is known as cholesterol efflux capacity [57]. HDL is also a heterogeneous lipoprotein with different particle sizes each with different cholesterol efflux capability [58]. HDL-2 and HDL-3 are the most studied classes of HDL, and they differ by size and functionality with HDL-2 showing the most cholesterol efflux potential [59]. n-3 PUFA has been reported to increase the concentration of larger and buoyant HDL-2 particles without affecting the total HDL cholesterol concentration [60], thereby increasing cholesterol efflux which has cardioprotective implication.
27.3.1.2 n-3 PUFA and the Regulation of Genes Involved in Lipid Metabolism
Abnormal concentration of lipids known as dyslipidaemia is a major independent risk factor of CVD [61]. Hypertriglyceridaemia is an independent risk factor for the development of CVD [62]. Fish oil has been shown to be potent at treating hypertriglyceridaemia [63]. It has been reported that 3–4 g/day EPA and DHA resulted in 25 % reduction of TG levels in normolipidaemic individuals and 35 % reduction in TG in individuals with hyperlipidaemia [31,35]. The mechanism underlying the TG-lowering effects of n-3 PUFA has been explained by the involvement of n-3 PUFA in the regulation of genes involved in lipid metabolism; such genes include SREBP, all forms of peroxisome proliferator-activated receptors (PPARs), retinoid X receptor-alpha (RXR-α) and LXR-α. The retinoid X receptor (RXR) heterodimerizes with LXR and regulates the activity of SREBP1-c by binding to the LXR response element in the SREBP1-c promoter region, thereby suppressing its expression [64]. SREBP1-c is the key controller of lipogenesis; its inhibition would downregulate the expression of fatty acid synthase and acetyl-CoA carboxylase, thereby preventing fatty acid synthesis required for TG synthesis. Another classic cardioprotective mechanism of n-3 PUFA in the reduction of circulating TG is by stimulating fatty acid β-oxidation. n-3 PUFA reduces TG concentration by increasing the β-oxidation of nonesterified fatty acids (NEFA), a substrate for TG synthesis [65]. n-3 PUFA also regulates the expression of PPARα which upregulates acetyl coenzyme A oxidase, a rate-limiting enzyme in fatty acid catabolism, which further stimulates β-oxidation [66].
27.3.2 Cardioprotective Effects of n-3 PUFA by Altering Membrane Dynamics and Production of Bioactive Lipids
Membrane fluidity is influenced by the incorporation of long-chain n-3 PUFA into membrane phospholipids [67,68], which could alter the functions of transmembrane proteins and their interaction with extracellular ligands [69]. These alterations indirectly affect signalling pathways and other physiological functions. Furthermore, the fatty acid on the sn-2 position of membrane phospholipids could be cleaved by the action of phospholipase A2 (PLA2) to produce a free fatty acid and lysophosphatidylcholine (LPC) which are both bioactive molecules [70]. The released 20-carbon AA and EPA from the membrane phospholipids undergo oxidation catalyzed by cyclooxygenases (COX-1 and COX-2) and lipoxygenases (LOX-5, LOX-12, LOX-15) to produce bioactive lipid intermediates and hormone-like substances known as eicosanoids [38]. AA is a precursor to series-2 prostaglandins and series-4 leukotrienes; these are generally proinflammatory, vasoconstrictive and pro-aggregatory and generally promote CVD [22]. On the other hand, series-3 prostaglandins and series-5 leukotrienes produced from EPA are anti-inflammatory and prevent the development CVD [23]. AA-derived eicosanoids include proinflammatory PGE2 (vasodilator), TXA2 (vasoconstrictor), PGI2 (platelet aggregator) and LTB4 (chemotactic factor), while EPA-derived eicosanoids include anti-inflammatory PGE3 (vasodilator) and PGI3 (inhibit platelet aggregator), and LTB5 and TXA3 are less active than AA-derived eicosanoids [14,71] (Fig. 27.1).
Cardiac arrhythmia is a major event of CVD, and this involves the electrophysiological control of cardiac muscle activities [72]. n-3 PUFA has been shown to control cardiac arrhythmia by regulating Na+, Ca2+, K+ channels and ion pumps [72]. n-3 PUFAs are relatively safe; however, they have been shown to influence platelet function and could affect the bleeding time. n-3 PUFAs increase the concentration of PG12 or prostacyclin, a vasodilator and platelet inhibitor [73]; this also leads to a reduced formation of the platelet activator TXA2 (11). Furthermore, n-3 PUFAs suppress the production of platelet-activating factor (PAF), also a platelet activator [73]; this consequently increases bleeding time and reduces ADP, epinephrine and collagen-stimulated platelet aggregation in individuals supplemented with n-3 PUFA [74]. Although n-3 PUFAs have been shown to affect bleeding time, the changes in bleeding time are within the normal range and are not associated with any bleeding complications. Studies that supplemented n-3 PUFA within the range of 3–6 g/day to healthy subjects and patients with cardiovascular diseases without any concomitant medication that prolongs bleeding time reported an increase in bleeding time, which was within the normal range [75]. Other studies that administered up to 6 g/day n-3 PUFA saw no significant difference in bleeding time [76,77].
DHA and EPA produced from membrane phospholipids can also be oxygenated to produce a novel class of inflammation-resolving compounds known as resolvins and protectins which are also cardioprotective [78]. The resolution of inflammation involves a decrease in proinflammatory mediators, clearance of leukocytes and the transition of the cells back to a non-inflammatory state [79]. Inflammation-resolving lipid mediators are produced based on the parent fatty acid and the presence or absence of aspirin [80]. The expression of COX-2 is upregulated during acute inflammation [81], and in the presence of aspirin, COX-2 is acetylated thereby inhibiting the formation of prostaglandins [80]. Acetylated COX-2 can catalyze a series of reactions using EPA and DHA leading to the formation of resolvins (resolution phase interaction products). Resolvins are oxygenated anti-inflammatory and immunoregulatory products of the metabolism of EPA and DHA. The resolvins and protectins elicit their beneficial effects in the picomolar and nanomolar range both in vivo and in vitro [82,83]. EPA is oxygenated to produce the E-series resolvins, while DHA produced resolvins or protectins of the D-series. Both the D- and E-series resolvins are formed by 15-LOX in leukocytes and aspirin-acetylated COX-2 in vascular endothelial cells [84], while protectins are formed from DHA by the oxygenation of 15-LOX in leukocytes and other cells [23,85]. Resolvins exhibit their anti-inflammatory properties by inhibiting the transmigration and infiltration of neutrophils mainly by initiating apoptosis [83] which is important in the resolution of inflammation. Protectins on the other hand prevent inflammation by blocking the recruitment and action of neutrophils, inhibiting the secretion of TNF-α and altering the activities of COX-2 in both in vivo and in vitro settings [86]. Protectin and resolvins are capable of resolving inflammations associated with atherosclerosis, mainly by downregulating the expression of inflammatory proteins such as MCP-1 and IL-8 [87].
The type of the released fatty acid from the membrane phospholipids depends on the dietary fatty acid composition. We have previously shown that consuming a diet high in n-3 PUFA alters the fatty acid composition of membrane phospholipids such as phosphatidylcholine (PC) and phosphatidylethanolamine (PE), thereby resulting in increased release of cardioprotective n-3 PUFA such as free EPA and DHA [42]. n-3 PUFAs have also been shown to cause an increase in the n-3 PUFA composition of lysophosphatidylcholine (LPC), which is also a product of the enzyme hydrolysis of membrane PC [42]. LPC is biologically active and regulates several metabolic and physiological pathways by acting as a signalling molecule [88]. LPC is generally considered atherogenic in nature [89]; however, the biological and metabolic functions of LPC have been suggested to depend on the acyl chain length and degree of unsaturation [90]. Fish oil intake was found to increase plasma and tissue concentrations of LPC enriched with EPA and DHA [42,90,91]. The consumption of fish oil in subjects with impaired glucose metabolism significantly increased plasma LPC-EPA [92], and LPC was found to enhance insulin secretion [93]. LPC with DHA is also known to possess anti-inflammatory properties with overall cardioprotective effect [88]. Furthermore, LPC containing n-3 PUFA has been shown to promote cholesterol efflux from macrophages consequently increasing the overall reverse cholesterol transport which has a cardioprotective implication [94]. Figure 27.2 summarizes the potential of n-3 PUFA-enriched LPC to act as an anti-atherogenic molecule. Overall, there is plenty of evidence that enrichment of membrane n-3 PUFA content has the ability to modify metabolic and physiological functions, thereby inducing cardioprotective effects.
27.3.3 Cardioprotective Effects of n-3 PUFA by Reducing Inflammation
Atherosclerosis is defined as the pathological thickening of the arterial wall as a result of invading white blood cells, which is the major underlying process for the development of CVD [95]. The development of atherosclerosis encompasses a cycle of inflammatory processes and is now recognized as an inflammatory disease [96]. Atherosclerosis develops from the oxidation of LDL chiefly by reactive oxygen species. The oxidized LDL triggers a sequence of inflammatory responses resulting in an increased expression of adhesion molecules such as vascular cell adhesion molecule 1 (V-CAM-1), intercellular adhesion molecule 1 (ICAM-1) and E-selectin by the endothelial cells. A chain of chemical reactions lead to the recruitment of monocytes which later differentiate in macrophages; these macrophages take up the oxidized LDL and become lipid laden to form foam cells. The foam cells continue to grow and rupture leading to a huge deposit of cholesterol and fatty streaks on the endothelial wall. More macrophages are then recruited upon rupture of the foam cells, and the cycle continues which could eventually lead to end-stage CVD [97] (Chap. 25).
Studies have reported the ability of n-3 PUFA to reduce the expression of adhesion molecules on macrophages [98] and endothelial cells [99], thereby preventing the atherosclerotic plaque progression. n-3 PUFAs also prevent CVD by maintaining vascular endothelial function. The adhesion molecules secreted by endothelial cells are actively involved in platelet adhesion and leukocyte recruitment during inflammation, which are major players in atherogenesis [23]. These adhesion molecules are activated by proinflammatory cytokines such as TNF-α, IL-6 and IL-8, and n-3 PUFA has been shown to decrease the expression of these cytokines.
The expression of adhesion molecules on the endothelium is also promoted by nuclear factor kappa-light-chain-enhancer of activated B cells (NF-kB), a proinflammatory transcription factor [100,101]. NF-kB is involved in a number of cellular responses including inflammation and immune response to infection. Activation of NF-kB leads to a sequence of reactions that promote inflammation and consequently atherogenesis. The activated form of NF-kB has been found in atherosclerotic vessel walls that promotes the formation of atherosclerotic lesion [102]. n-3 PUFA downregulates the expression of NF-kB to suppress inflammation [103]; this reduces the markers of atherosclerosis and enhances vascular function [104]. n-3 PUFA has also been found to impede the translation of genes involved in inflammation by inhibiting NF-kB. Furthermore, EPA was found to inhibit the expression of TNF-α, a classic proinflammatory cytokine by preventing the movement of NF-kB into the nucleus [105]. NF-kB also upregulates the expression of inflammatory cytokines such as IL-6, IL-2 and TNF-α; n-3 PUFAs have been shown to reduce these cytokines. The expression of PPARs, an important class of nuclear receptor proteins involved in the regulation of inflammation and lipid metabolism, has also been shown to be directly regulated by n-3 PUFA [106]. PPAR forms heterodimer with retinoid X receptor (RXR); the PPAR/RXR heterodimer binds to the peroxisome proliferator response element (PPRE) on the target gene to elicit its regulatory role [23]. PPARα and PPARγ inhibit NF-kB, thereby blocking the production of potent inflammatory cytokines. PPARα has also been shown to exhibit its cardioprotective effect by blocking the production of cellular adhesion molecules, leading to a significant reduction of inflammation [106]. Moreover, as mentioned previously, n-3 PUFA can also alleviate inflammation resulting from atherogenesis by producing anti-inflammatory eicosanoids and docosanoids from EPA and DHA, respectively [107].
27.4 Potential Reasons for Controversies in the Cardioprotective Effects of n-3 PUFA
There are numerous reports on the beneficial health effects of n-3 PUFA; however, there are also controversial reports on the cardioprotective efficacy of n-3 PUFA. A recent systematic review and meta-analysis on the association between n-3 PUFA supplementation and risk of CVD revealed that n-3 PUFA was not associated with lower mortality from cardiovascular events [108]. Furthermore, a recent study reported no significant effect on markers of CVD after 6-year supplementation with n-3 PUFA [109]. These controversies could be due to differential effects of n-3 PUFA based on sex, dose, duration of exposure and the type of n-3 PUFA used.
27.4.1 Sex, Age and the Cardioprotective Effects of n-3 PUFA
The most characterized factor affecting lipid metabolism is sex; this has been ascribed to a clear distinction in hormonal regulation between male and female [110]. Research evidence suggests that sex also plays a vital role in the development of CVD; it has been reported that men and women differ significantly in their circulating blood lipids and predisposition to CVD [111] (Chap. 21). Plasma TC has been shown to increase with age peaking at 50–59 years in men and at 60–69 years in women [112]; these sex-specific differences in blood lipid parameters have also been reported for plasma HDL cholesterol and TG [112]. The female hormone, oestrogen, is involved in lipid metabolic pathways; it is also responsible for the rapid transport of fat in women compared to men, and this partly accounts for the gender-specific risk of CVD [113]. These sex-specific differences have also been shown to affect the metabolism of n-3 PUFA, which would explain the overall sex differences in cardiovascular effects of n-3 PUFA. Studies have reported a higher tissue accretion of DHA in females compared to male [114,115], supported by the higher conversion rate of ALA to longer-chain EPA and DHA in women [114]. This is explained mechanistically by the sex-specific effect of the liver desaturase enzyme responsible for the conversion of ALA to EPA and DHA [115]. Interestingly oestrogen has also been shown to affect tissue distribution of n-3 PUFA with females showing a higher accretion which further explains the differences observed between male and female [116]. Although controversial, the role of oestrogen in LDL metabolism has been reported; oestrogen decreased the circulating concentration of atherogenic LDL [117]. We have also observed an interaction between n-3 PUFA and sex in a rodent model, where female mice showed a lower concentration of plasma LDL cholesterol concentration in response to high-n-3 PUFA diet compared to male mice [118]. There are also sex differences in the concentration of HDL particles between men and women [113]. More importantly, cholesterol efflux capacity is sex specific and is higher in males than in females in response to high-n-3 PUFA diet [118]. The mechanism underlying the sex-specific effects of n-3 PUFA has been explained by the interaction between PPARα and oestrogen receptor. Studies have shown a stimulatory relationship between PPARα and oestrogen receptor, which is affected by n-3 PUFA [119,120]. There are also evidences of the sex-specific effect of PPARα on the regulation of cholesterol metabolism [121].
The effect of sex in the development of CVD cannot be overemphasized; however, beyond sex differences comes age. The prevalence of CVD is different between male and female, and this has also been shown to be affected with age [122]; this has been linked to age-dependent variation in cholesterol concentration between male and female [123]. The plasma TC concentration increases with age [124] mainly because of the age-dependent decrease in the catabolism of cholesterol [124]. Furthermore, males and females have comparable plasma HDL cholesterol concentration at puberty after which the differences become apparent as they age [125]. There are also documented age effects on plasma concentration of TG concentration [122]. Recent evidence also supports the effect of age and n-3 PUFA on the concentration of plasma lipids and lipoproteins [118] (Chap. 21).
27.4.2 Dose and the Cardioprotective Effects of n-3 PUFA
To get the desired cardioprotective benefits from n-3 PUFA, it is imperative to administer the appropriate dosage. The recent systematic review and meta-analyses that discredited the cardioprotective effects of n-3 PUFA [108] considered studies that used a lower than the recommended dosage of n-3 PUFA; the mean intake of EPA and DHA in their study was approximately half the recommended dosage of 3 g/day to reduce the risk of CVD. The beneficial effect of n-3 PUFA on cardiovascular events has been shown to be dose dependent. Moertl et al. found a 2.5 % improvement in left ventricular ejection fraction (LVEF) in patients with coronary heart failure (CHF) after administering 1 g/day n-3 PUFA for 3 months; however, a 5.5 % improvement was recorded with a higher dose of 4 g/day of n-3 PUFA [126]. In the same cohort, only higher doses were seen to be effective at resolving inflammation and alleviating endothelial dysfunction, further emphasizing the importance of dose in the health benefits of n-3 PUFA [126]. Furthermore, studies have also reported the beneficial effect of n-3 PUFA dosage greater than 1 g/day at treating endothelial dysfunction [127]. To obtain a full cardioprotective effect of n-3 PUFA, a dosage in excess of the currently recommended 1 g/day will be required. The immunomodulatory effects of n-3 PUFA has also been shown to be dose dependent, with observable benefits within the range of 1.65 and 3.3 g/day [128]. The importance of dosage in the health benefits of n-3 PUFA has also been corroborated by animal studies with significant improvement from cardiovascular events observed at a higher dosage [129]. Several clinical trials have reported the beneficial effects of consuming fish and n-3 PUFA supplements, and a different dosage has been shown to be effective. In a clinical trial, subjects who were asked to consume 200–400 g oily fish per week or the equivalent of fish oil capsules (EPA 180 mg and DHA 120 mg) saw significant cardioprotective effects [27]. In another study, individuals who received approximately 882 mg of EPA and DHA (1:2) had up to 20 % reduction in risk of CVD [130] compared to those without n-3 PUFA supplements [131]. Analyses of prospective cohort studies have shown that the consumption of 25–500 mg/day EPA and DHA is sufficient to reduce the risk of CVD [132]; however, another study reported that the upper limit of 500 mg/day provided the most beneficial effect [133] and that even greater intake will confer additional protection [134]. It was also reported that 4 g/day reduced TG concentration by 25–30 %, and a dose response relationship was also observed [31]. Another study of 42 participants revealed a 45 % decrease in TG after supplementing patients with 4 g/day n-3 PUFA [135]. The cardioprotective benefits of n-3 PUFA have been well documented; however, the optimal dose remains to be established. There is no firm recommended dietary intake for n-3 PUFA; however, different international organizations have dietary recommendations (Table 27.1). The effective dose of n-3 PUFA to treat an underlying CVD ranges from 3 to 5 g/day, which can only be obtained by supplements [136].
27.5 n-3 PUFA, Epigenetics and CVD
It has been clearly stated that genetics and environment are the key players in the development of CVD; however, intertwined between the two is epigenetics. Over the past couple of years, the area of system biology has experienced an “-omic” revolution. This continues to shape our understanding of molecular biology with technologies in genomics, transcriptomic, proteomics, metabolomics and lipidomics. Epigenetics refers to the study of changes resulting from gene expression caused by factors other than changes in the genome. These changes result in long-term modification in the genome resulting in an alteration in transcriptional regulation of DNA [137]. In 1940, Conrad Waddington defined epigenetics as “the causal interaction between genes and their products, which brings the phenotype into being” [138]. Epigenetic changes occur mainly by DNA methylation and chromatin remodelling. Chromatin is remodelled through DNA methylation at the CpG site, converting cytosine to 5-methyl cytosine. DNA methylation reduces the transcriptional activity of the DNA, which results in the silencing of some genes. Noncoding RNAs known as microRNAs (miRNA) have also been shown to be involved in epigenetic modification. Epigenetic regulation has made its way into lipid metabolism, where a number of genes and proteins involved in lipid metabolism are capable of being subjected to epigenetic modification. PPAR, a protein with activities central to lipid metabolism, has been shown to be regulated by DNA methylation [139]. Acyl-CoA oxidase, the rate-limiting enzyme of the peroxisomal β-oxidation pathways, and directly regulated by PPARγ [140], was also shown to be regulated by DNA methylation [139]. LXR-α promoter region has been identified as a target for DNA hypermethylation during protein restriction, and its transcription is dependent on DNA methylation. Cholesterol metabolism has been shown to be regulated by miR-33 regulatory miRNA found in the gene coding for sterol regulatory element-binding factor-2 (SREBF-2), a vital regulator of cholesterol transport [141]. The miR-3 was also shown to repress the expression of ABCA1 gene, thereby attenuating cholesterol efflux via apolipoprotein-A1 [141]. Recent evidence suggests that n-3 PUFA could influence epigenetic modification. The role of DHA in one carbon metabolism and epigenetics has been reported [142]. Folic acid and vitamin B12 are the major players in one carbon metabolism which produces S-adenosyl methionine (SAM), a methyl group donor [143]. There are studies suggesting that n-3 PUFA in addition with folic acid and vitamin B12 increased plasma homocysteine which could affect DNA methylation, in schizophrenic individuals [144]. It is proposed that n-3 PUFAs alter epigenetic DNA methylation where reduced dietary consumption of DHA decreases the concentration of PE [145], a chief methyl acceptor to form PC. A decrease in PE results in excess methyl group available for DNA methylation and altered expression of genes [146]. Furthermore, n-3 PUFA was shown to affect the epigenetic status of the hepatic fad2 gene that codes for δ-6 desaturase, the rate-limiting enzyme in the synthesis of AA and DHA in C57BL/6 mice [147]. Although there are no available data in the literature that link the epigenetic regulation of n-3 PUFA to CVD, it would be plausible to think that there is a direct relationship between epigenetic modifications and the cardioprotective roles of n-3 PUFA, considering the fact that most of the genes and proteins regulated by n-3 PUFA have been shown to be targets of epigenetic modification. However, studies could be designed to ascertain the role of n-3 PUFA in epigenetic regulation.
< div class='tao-gold-member'>
Only gold members can continue reading. Log In or Register a > to continue
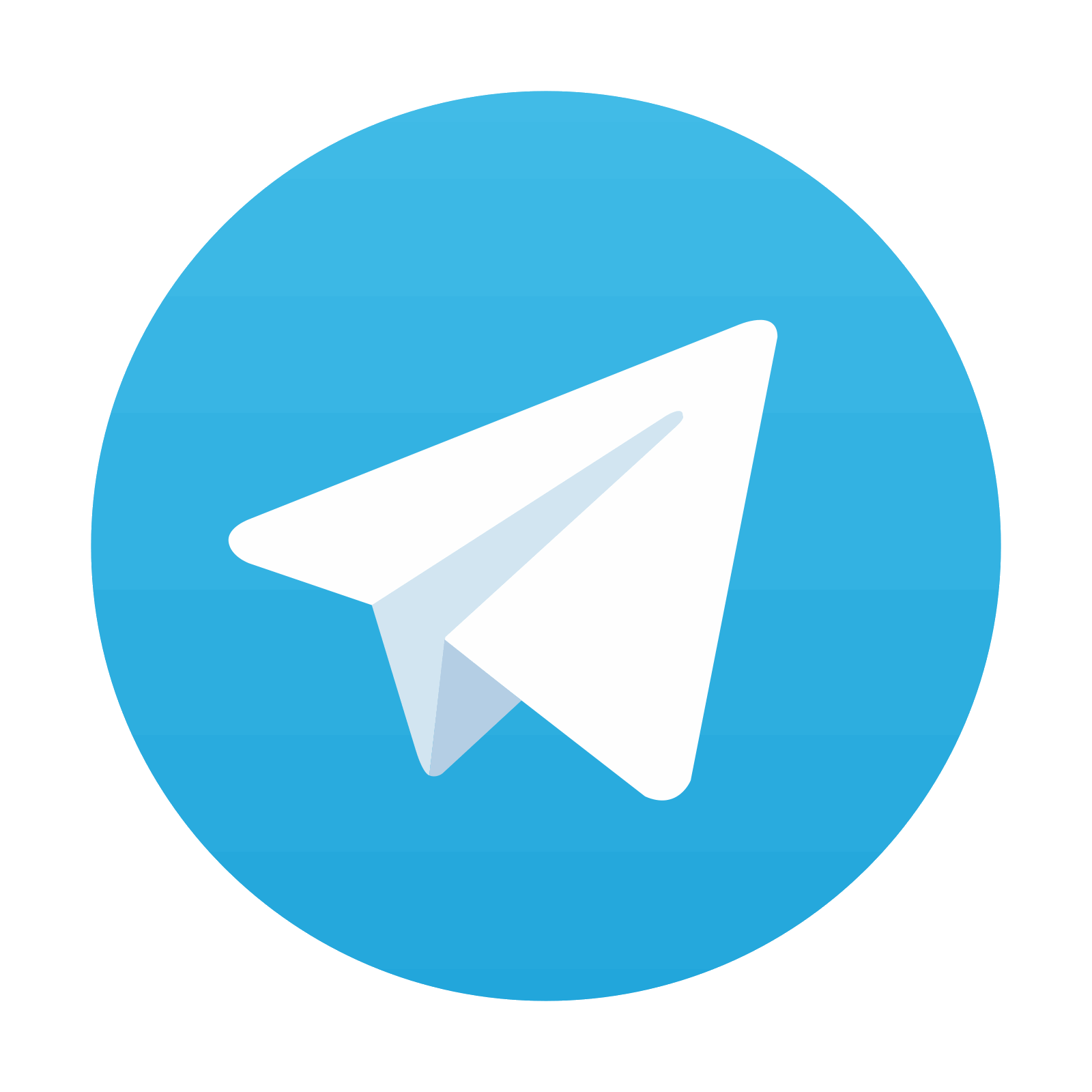
Stay updated, free articles. Join our Telegram channel
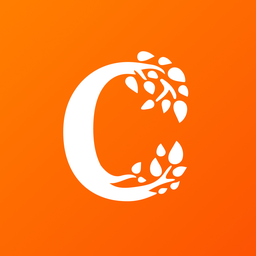
Full access? Get Clinical Tree
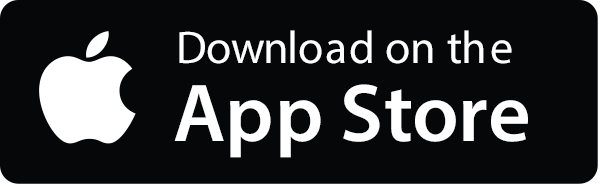
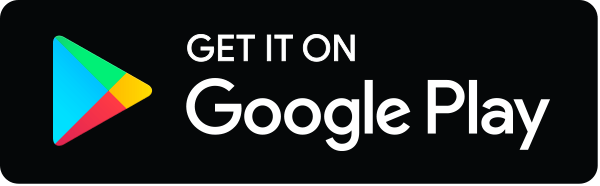