The term “cardiomyopathy” is used to indicate myocardial dysfunction in the absence of an obstructive lesion or sustained hypertension. Cardiomyopathy can occur either in isolation or as a manifestation of a multisystem disease. Neonates who have an unrecognized cardiomyopathy may come to medical attention with a life-threatening decompensation associated with an otherwise minor illness, such as a viral upper respiratory infection. Alternatively, evidence of cardiomyopathy may be noted on an echocardiogram performed for evaluation of an unrelated problem. Other conditions cause ventricular dysfunction but may not have a long-term impact on cardiac muscle function, such as myocarditis or anomalous origin of the left coronary artery from the pulmonary artery, and will be discussed at the end of the chapter.
Mutations in multiple genes encoding proteins of the sarcomere, cytoskeleton, sarcoplasmic reticulum, nucleus, and cell membrane of the myocardial cell are now known to cause cardiomyopathy (Figure 9-1). More information regarding the structure and function of these proteins is presented in Chapter 2.
FIGURE 9-1.
Myocyte cytoarchitecture. Various forms of cardiomyopathy may result from mutations in genes encoding multiple proteins within the cardiac myocyte. Different mutations in the same gene may cause different forms of cardiomyopathy. Abbreviations: EYA4, eyes absent homolog 4; MLP, cardiac LIM domain protein; MyBPc, myosin binding protein C; T-cap, telethonin; ZASP, muscle LIM-binding protein 3 (cypher).

Classification of cardiomyopathies is challenging and has evolved as new information has become available regarding causation. Classification based on phenotype (ventricular morphology and physiology) is practical because these characteristics are typically defined at the initial evaluation of the patient (Table 9-1). Phenotypic groups can be subdivided based on etiology (Tables 9-2, 9-3, 9-4). Unfortunately, specific causes of cardiomyopathy can present with different phenotypes in different patients or be associated with different phenotypes during the evolution of the disease in a specific patient, making classification by phenotype imperfect.
OMIM number | |
---|---|
Familial, unknown gene | |
Myofilament (sarcomeric) mutation | |
Thick filament | |
β-myosin heavy chain | 160760 |
Ventricular regulatory myosin light chain | 608758 |
Ventricular essential myosin light chain | 608751 |
Intermediate filament | |
Cardiac myosin-binding protein C | 115197 |
Thin filament | |
Cardiac troponin T | 115195 |
Cardiac troponin C | 613243 |
Cardiac troponin I | 613690 |
α-tropomyosin | 115196 |
α-cardiac actin | 612098 |
Z-disc mutation | |
Cardiac LIM domain protein (cysteine- and glycine-rich protein 3) | 612124 |
AMP-activated protein kinasea | 600858 |
Storage diseases (Table 9-7) | |
Fatty acid oxidation disorders (Table 9-5) | |
Respiratory chain disorders (Table 9-6) | |
Congenital disorder of glycosylation, type Ia (phosphomannomutase-2) | 212065 |
Neuromuscular disease | |
Malformation syndrome (Noonan, Beckwith Wiedemann, Costello, LEOPARD, cardio-facial-cutaneous)a | |
Congenital heart disease associated with ventricular outflow tract obstruction | |
Systemic hypertension | |
Infant of diabetic mother |
OMIM number | |
Familial, unknown gene | |
Myofilament (sarcomeric) mutations | |
Giant filament | |
Titin | 604145 |
Thick filament | |
β-myosin heavy chain | 613252 |
Thin filament | |
Cardiac troponin T | 601494 |
Cardiac troponin C | 611879 |
Cardiac troponin I | 611880 |
α-tropomyosin | 611878 |
α-cardiac actin | 613424 |
Z-disc mutations | |
LIM domain-binding protein 3 (ZASP, cypher) | 601493 |
Telethonin | 607487 |
α-actinin 2 | 612158 |
Cardiac LIM domain protein (cysteine- and glycine-rich protein 3) | 607482 |
Nexilin | 613122 |
Cytoskeletal gene mutations | |
Dystrophina | 302045 |
Metavinculin | 611407 |
Sarcoglycan complex | 606685 |
Intercalated disc protein mutations | 612877 |
Desmoglein-2 | |
Sarcoplasmic reticulum mutations | |
Phospholamban | 609909 |
Nuclear membrane mutations | |
Lamin A/C | 115200 |
Emerina | 300384 |
Transcriptional coactivator mutations | |
Eyes absent homolog 2a | 605362 |
Voltage-gated channel mutations | |
Cardiac sodium channel SCN5A | 601154 |
Fatty acid oxidation disorders (Table 9-5) | |
Respiratory chain disorders (Table 9-6) | |
Nonfamilial | |
Myocarditis | |
Kawasaki diseasea | |
Thyroid diseasea | |
Drugs (anthracyclines, emetine, lead)a | |
Peripartum cardiomyopathya | |
Nutritional (thiamine, carnitine, selenium, hypophosphatemia, hypocalcemia) | |
Tachycardia-induced cardiomyopathy | |
Transplant rejection | |
Myocardial insufficiency—ALCAPA, tachyarrhythmias |
OMIM number | |
---|---|
Familial, unknown gene | |
Sarcomeric protein mutations | |
Troponin I | 115210 |
Troponin T | 612422 |
α-cardiac actin | |
β-myosin heavy chain | |
Myosin light chain | |
Nonsarcomeric mutations | |
Desminopathya | 609578 |
Amyloidosisa | |
Sclerodermaa | |
Hemachromatosisa | |
Glycogen storage disease (Table 9-7) | |
Endomyocardial fibrosis | |
Hypereosinophilic syndromea | |
Idiopathica | |
Chromosomal | |
Drugs (serotonin, methysergide, ergotamine, busulfan)a | |
Radiation toxicitya |
The clinician should first perform a careful assessment of the disease phenotype (cardiac and extracardiac) and then proceed in a logical manner to elucidate the etiology. Genetic defects and abnormal proteins have been identified for many forms of cardiomyopathy that were thought previously to be idiopathic. Identification of a specific cause will facilitate genetic counseling and may lead to specific therapy and possibly to prevention in family members. This chapter reviews the varied clinical presentations and many associated disorders and provides a rational approach to the array of available diagnostic tests and biochemical assays. Many forms of cardiomyopathy do not present in the neonatal period; as such, they are included for informational purposes in tables but are not discussed further. The reader is referred to several excellent recent reviews listed in “Suggested Readings.”
Hypertrophic cardiomyopathy is characterized by a hypertrophic, nondilated left ventricle in the absence of systemic disease (eg, hypertension) or structural abnormalities (eg, aortic stenosis) that can result in left ventricular hypertrophy. The hypertrophy may be asymmetric, affecting the interventricular septum more than the left ventricular free wall. In infants, the right ventricle may also be involved.
This phenotype may be present in many conditions discussed in the following text, including idiopathic, familial hypertrophic cardiomyopathy, inborn errors of metabolism (usually involving multiple organs), genetic and other malformation syndromes, neuromuscular disease, and infants of diabetic mothers (Table 9-2). The age of presentation and clinical course are highly dependent on etiology.
The Pediatric Cardiomyopathy Registry has published information regarding epidemiology and outcomes in groups of children with the phenotype of hypertrophic cardiomyopathy. Of the 855 patients from North America, 328 (38%) presented before 1 year of age. Of these, 15% had an inborn error of metabolism, 15% had a malformation syndrome, 1% had neuromuscular disease, and 69% were unclassified or idiopathic. Children with an inborn error of metabolism or malformation syndrome (mostly Noonan syndrome) presenting with hypertrophic cardiomyopathy before 1 year of age have a particularly poor prognosis, with a 5-year survival after diagnosis of 26% and 66%, respectively. The 1-year survival from the time of diagnosis in patients in the idiopathic group who were diagnosed at younger than 1 year of age was 86% compared with 99% in those diagnosed older than 1 year of age. However, no difference in annual mortality was observed between these age-groups for those patients who survived beyond 1 year of age. Similar outcomes were reported in a registry of Australian patients. Other presenting findings associated with a worse prognosis were lower weight Z score and the presence of congestive heart failure.
In typical familial hypertrophic cardiomyopathy, histologic examination discloses cellular and myofibrillar disarray, interstitial fibrosis, and coronary arterial abnormalities. The genetic defect is heterogeneous; hundreds of different mutations, mostly missense, are described in more than 23 genes involving myofilament and Z-disc proteins. A smaller number have been substantiated through cosegregation and linkage analysis studies (Table 9-2 and Figure 9-1). Defects in myofilament (sarcomeric) proteins show an autosomal dominant pattern of inheritance and account for about 60% of cases. Mutations in β-myosin heavy chain (20% to 30%) and myosin-binding protein C (30% to 40%) are most common. Most disease-causing mutations are unique to a single family. Phenotypic expression within families is highly variable and is influenced by modifier genes and/or environmental factors (epigenetic modifications).
The precise mechanism of how a gene defect leads to the anatomic and functional abnormalities observed in these patients is poorly defined and likely varies among the different defects. Possibilities include abnormal calcium cycling, increased or decreased sarcomeric calcium sensitivity, altered biomechanical stress sensing, and impaired ventricular energy homeostasis.
Clinical genetic testing is currently available for 9 to 18 genes, depending on the laboratory, and is useful primarily for diagnosis rather than for predicting outcome or for guiding therapy. Of those patients who undergo testing, a mutation is identified in 40% to 60% of sporadic and familial cases when testing is performed for these genes (http://www.genetests.org). Genetic testing may not be covered by an individual patient’s health insurance plan. Of the 855 patients with hypertrophic cardiomyopathy in the Pediatric Cardiomyopathy Registry, 74% had no associated illness, no family history, and no defined genetic defect. Given the lack of reproducible phenotype–genotype correlation, the results of genetic testing may not impact patient management. In contrast, genotyping of children of affected individuals may be helpful in determining who needs repeated screening for disease.
Left ventricular hypertrophy associated with familial hypertrophic cardiomyopathy is often not present until adulthood. However, if hypertrophy is present in infancy, this may be severe enough to produce obstruction of right or left ventricular outflow. These patients have a high probability of mortality in the first year of life, usually because of congestive heart failure. The usual medical therapies for congestive heart failure are contraindicated in these patients. Positive inotropic agents and diuretics may increase outflow tract obstruction and symptoms. Administration of β-blocking agents is the most widely accepted therapy. Oral administration of calcium channel blockers, such as verapamil and nifedipine, may also be beneficial. Given the poor prognosis for many of these infants, heart transplantation should be considered early in the course.
Dilated cardiomyopathy is characterized by dilation of the ventricles and decreased ventricular systolic function in the absence of abnormal loading conditions (eg, valve disease) or other conditions that can cause global systolic dysfunction, such as severe anemia, hypoglycemia, or acidosis. This is the most common phenotype of cardiomyopathy and is also the most common reason for cardiac transplantation in children. According to data from the Pediatric Cardiomyopathy Registry, the incidence of dilated cardiomyopathy is higher in infants younger than 1 year of age than in older children (4.40 vs. 0.34 per 100,000 per year; P < .001).
A number of conditions are known to cause dilated cardiomyopathy (Table 9-3). The etiology for 591 children younger than 1 year of age in the Pediatric Cardiomyopathy Registry was unknown (idiopathic) in 78%, myocarditis in 11%, inborn error of metabolism in 5%, familial in 4%, malformation syndrome in 1.7%, and neuromuscular disease in <0.1%.
Hereditary factors have been recognized recently to play a role in the etiology of idiopathic dilated cardiomyopathy in an increasing percentage of patients. In newly diagnosed adult patients, dilated cardiomyopathy can be identified in up to 20% to 35% of screened first-degree relatives, and these patients are then classified as having familial dilated cardiomyopathy. The pattern of inheritance is autosomal dominant in about 90% of adult cases. Penetrance and expression are frequently incomplete and age dependent. Affected family members with certain mutations may show different phenotypic patterns of cardiomyopathy. In some studies, at least 80% of mutation carriers under the age of 20 years are clinically well. Familial disease is much less common in young infants, but screening of all first-degree relatives by electrocardiography and echocardiography is recommended when the diagnosis is made.
Familial dilated cardiomyopathy is genetically heterogeneous (at least 50 genes) and is caused by mutations in genes encoding proteins of the nucleus, sarcoplasmic reticulum, sarcolemma, mitochondria, and the cytoskeleton (Table 9-3 and Figure 9-1). In general, these mutations result in diminished force generation or alterations in mechanotransduction or myocyte signaling. Mutations in the large sarcomeric protein titin account for about 25% of adult cases, but none of the other known mutations account for more than a few percent of cases. Clinical genetic testing is available for a variety of genes and will identify a pathologic mutation in 30% to 40% of patients. Most of the identified mutations are unique to the patient or family. Screening of family members is made difficult by the fact that genetic variants thought to be disease causing are often identified in the absence of clinical disease.
Of particular interest is the fact that different mutations in the same genes for sarcomeric proteins that are well known to be associated with hypertrophic cardiomyopathy are also associated with dilated cardiomyopathy. This suggests that the precise location of the mutation on the gene and the resulting consequences on protein structure and function play an important role in determining cardiomyopathy phenotype. The myofiber disarray seen in hypertrophic cardiomyopathy is absent in dilated cardiomyopathy caused by mutations in the same sarcomeric proteins. Interestingly, such mutations tend to increase calcium sensitivity of the cardiac myofilaments in hypertrophic cardiomyopathy, yet they tend to decrease calcium sensitivity in dilated cardiomyopathy. Further studies are needed to understand the molecular mechanisms by which these mutations result in alterations in calcium sensitivity and the pathways that lead to a dilated or hypertrophic phenotype.
Young infants with dilated cardiomyopathy typically present with signs and symptoms of congestive heart failure that should be treated using standard therapy (see Chapter 11). Age <1 year at diagnosis (especially <4 weeks of age), a family history of cardiomyopathy, and the severity of ventricular dysfunction are all independently associated with a higher risk of transplantation or death. Overall, the 5-year transplantation-free survival of children with dilated cardiomyopathy is about 50%. The need for transplantation is highest in children who have a familial form of dilated cardiomyopathy.
Restrictive cardiomyopathy is the rarest form of cardiomyopathy in the developed world, representing 2% to 3% of cardiomyopathies diagnosed in patients less than 18 years of age. Ventricular volume is normal or reduced, whereas atrial volume is usually markedly increased in the absence of congenital valve defects. Ventricular filling is impaired, and systolic function is normal or only minimally diminished. The left ventricular wall thickness is normal, but the restrictive phenotype can overlap with hypertrophic cardiomyopathy if both ventricular hypertrophy and a restrictive physiology are present.
Multiple conditions are associated with the restrictive phenotype (Table 9-4). Worldwide, the most common etiology of restrictive cardiomyopathy is a condition known as endomyocardial fibrosis, which is estimated to affect 10 million people, occurring most commonly in children and adolescents in tropical and subtropical regions, especially in Africa. The etiology is poorly defined, and genetic, autoimmune, dietary, and environmental factors may play a role. Fibrosis of the endocardium involves primarily the left ventricular apex, the mitral valve apparatus, and the right ventricular apex. Clinical manifestations vary with the site of involvement.
In the developed world, a specific etiology for restrictive cardiomyopathy cannot be identified in most cases. As described in the section above for both hypertrophic and dilated forms of cardiomyopathy, multiple mutations in both sarcomeric and nonsarcomeric proteins are associated with the phenotype of restrictive cardiomyopathy, and familial forms occur (Table 9-4). Additionally, some of the mutations are noted to cause both hypertrophic and dilated phenotypes in some family members.
Patients often present with respiratory complaints that are most prominent at times of exertion. The overall prognosis is poor and sudden cardiac death is common even in patients with only mild symptoms. Cardiac transplantation provides definitive therapy.
Noncompaction of the left ventricle is characterized by deep trabeculae with deep intertrabecular recesses involving most commonly the left ventricular apex and lateral wall, progressive impairment of ventricular function, and early death. Isolated forms may be inherited and are associated with mutations in a number of genes, many of which are known also to cause cardiomyopathy with hypertrophic or dilated phenotypes. This morphologic pattern is seen sporadically in association with a large number of disorders, including mitochondrial myopathies, Barth syndrome, neuromuscular disease, and various forms of congenital heart disease. In newborn infants, noncompaction is thought to be caused by a developmental arrest of unclear etiology resulting in insufficient compaction of the noncompacted myocardium during embryogenesis. The exact cause of the developmental arrest is not clear and is likely caused by different mechanisms in patients with different genetic disorders. In children, noncompaction cardiomyopathy is variably associated with ventricular hypertrophy, ventricular dilation, decreased systolic function, and arrhythmias. The risk of death or transplantation is highest in patients with ventricular dysfunction or arrhythmias and in those presenting in infancy.
A wide variety of disease entities are associated with cardiomyopathy. Many cause dilated cardiomyopathy, but others lead to hypertrophic, noncompaction, or restrictive phenotypes. Below is a presentation of some of the many causes.
As noted above, many genetic mutations of various components of the myocardial cell are specifically associated with cardiomyopathy, and the phenotype and expression can vary within the family. In addition to these myocyte-based genetic causes of familial cardiomyopathy, certain genetic syndromes are associated with cardiomyopathy unrelated to myocardial cell mutations. Some are associated with known inborn errors of metabolism (eg, Pompe disease and Barth syndrome) and will be discussed below. Malformation syndromes, such as Noonan syndrome, have a known genetic defect, but the etiology of the cardiomyopathy is unknown; these conditions are discussed in Chapter 15.
These disorders, although relatively rare, can cause catastrophic complications, including sudden death (Table 9-5). Arriving at a correct diagnosis is imperative because the prognosis is often favorable once appropriate treatment is provided. A working knowledge of these disorders is essential for anyone caring for young infants because a high index of suspicion is often necessary to recognize patients who have these defects.
OMIM number | |
---|---|
Defects in carnitine-dependent transport of long- and very long chain fatty acids | |
Systemic primary carnitine deficiency (carnitine uptake defect) (D) | 212140 |
Carnitine-acylcarnitine translocase deficiency (H) | 212138 |
Infantile carnitine palmitoyltransferase (CPT) II deficiency (H,D) | 608836 |
Defects in fatty acid β-oxidation | |
Very long chain acyl-CoA dehydrogenase (VLCAD) deficiency (H, D) | 201475 |
Medium-chain acyl-CoA dehydrogenase (MCAD) deficiency | 201450 |
Isolated long-chain 3-hydroxyacyl dehydrogenase (LCHAD) deficiency (H,D) | 609016 |
Trifunctional protein deficiency | 609015 |
Multiple acyl-CoA dehydrogenase deficiency (glutaric acidemia type 2) | 231680 |
Fats are an important source of fuel in the body and are the only substrate for oxidation during fasting or increased energy demand after depletion of hepatic glycogen. Decreasing blood glucose concentration causes lipid mobilization from fat stores. Stored fat is broken down into short-, medium-, long-, and very long chain fatty acids. Although skeletal and cardiac muscles metabolize fatty acids to generate ATP, the brain cannot metabolize fatty acids directly; ketone bodies synthesized by the liver are used for cerebral energy production.
The heart is critically dependent on adequate energy generation. The fetal heart relies on anaerobic glycolysis and lactate as energy sources. Among the many adjustments necessary during the transition to extrauterine existence is the use of fat as the main energy substrate for the heart. Up to 80% of the energy for cardiac function is produced by fatty acid oxidation in the mature heart.
Fatty acid oxidation, the process by which ATP is generated, occurs only within the mitochondria (Figure 9-2). A specific transporter facilitates movement of long- and very long chain fatty acids across the cell membrane into the cytosol. Long- and very long chain fatty acids are then activated by binding to coenzyme A (CoA) to form fatty acyl-CoA. This complex can then cross the outer mitochondrial membrane. At this point, the enzyme carnitine palmitoyl transferase I (CPTI) catalyzes transfer of the fatty acid from CoA to carnitine, forming acylcarnitine. The activity of this enzyme in the liver increases during fasting, which preferentially directs fatty acids to the liver. Acylcarnitine is then transferred across the inner mitochondrial membrane by an enzyme called carnitine acyl translocase. Once inside the mitochondria, the enzyme CPTII transfers the fatty acid from carnitine back to CoA. Short- and medium-chain fatty acids move across the plasma membrane (sarcolemma) and the outer and inner mitochondrial membranes without the aid of the carnitine-dependent transporter. Within the mitochondria, all fatty acids undergo β-oxidation, forming acetyl CoA (Figure 9-2). Acetyl CoA is metabolized by the tricarboxylic acid (Krebs) cycle, producing electrons that pass through the respiratory chain and generating ATP through oxidative phosphorylation (see following text). In fasting states, when glucose is relatively unavailable, acetyl CoA is metabolized to ketone bodies in the liver. Ketone bodies then circulate in the blood, where they are used preferentially by the brain for energy generation during fasting states.
FIGURE 9-2.
Oxidation of fatty acids. The trifunctional protein is composed of the enoyl-CoA hydratase, the long-chain 3-hydroxyacyl dehydrogenase (LCHAD) enzymes, and the 3-ketoacyl-CoA thiolase enzymes. Abbreviations: CPT, carnitine palmitoyl transferase; LCAD, long-chain acyl-CoA dehydrogenase; MCAD, medium-chain acyl-CoA dehydrogenase; SCAD, short-chain acyl-CoA dehydrogenase; TCA, tricarboxylic acid.

Carnitine plays a pivotal role in fatty acid oxidation. Carnitine can be obtained from the diet but is also synthesized from lysine and methionine in the liver, kidney, and brain but not in muscle. Carnitine is actively transported across the cell membrane by a carrier-mediated transport process. The carnitine concentration within the myocardial cell is 40 to 100 times above the concentration in plasma.
Disorders of fatty acid oxidation include abnormalities in carnitine-dependent transport or in mitochondrial β-oxidation of fatty acids. Defects may occur in any of the enzymes or transport proteins involved in fatty acid oxidation. Accumulation of substrates proximal to the specific enzyme defect explains many clinical findings in these patients:
Hypoketotic hypoglycemia—Partial oxidation of fatty acids by the liver produces ketones. Patients who have these defects cannot produce ketones, and therefore glucose becomes the only available fuel. During times of stress, for example, fasting, glycogen, and glucose stores become depleted, and hypoglycemia develops.
Encephalopathy or Reye-like syndrome—The brain is completely dependent on glucose and ketone bodies for energy generation. Neurological dysfunction results from failure to deliver these substrates to the brain during fasting.
Hepatic dysfunction and hyperammonemia—Hepatic dysfunction results from the toxicity of accumulating metabolites and ammonia concentrations increase because acetyl CoA is required for hepatic synthesis of urea.
Organ steatosis—Free fatty acids, which are released during fasting but cannot be metabolized, are often stored as triglycerides in various tissues. This may result in a hypertrophic or dilated cardiomyopathy, fatty liver, and/or lipid storage myopathy.
Abnormal urine organic acids—Fatty acids within the mitochondria that cannot undergo β-oxidation can be diverted to the endoplasmic reticulum for omega oxidation, which generates dicarboxylic acids and 3-hydroxydicarboxylic acids. These are excreted in the urine in an amount equal to or greater than the amount of ketones when the patient is fasting. Abnormal urine organic acids are generally not seen in patients who have defects involving the transport of fatty acids into the mitochondria.
Decreased plasma carnitine concentration—Carnitine concentrations are decreased in patients with many of these disorders, but this is a primary defect only in patients who have carnitine transporter deficiency. Carnitine concentrations are <5% of normal in these patients. In most other patients, carnitine deficiency (10% to 50% of normal) is secondary. Free fatty acids that accumulate because of the metabolic block are toxic, so excess fatty acids usually are conjugated to carnitine and glycine. These acylcarnitines compete with free carnitine during reabsorption in the renal tubules. Free carnitine is excreted preferentially because the affinity of the renal carnitine transporter is higher for longer chain-length acylcarnitines. Thus decreased carnitine concentrations are secondary, and the ratio of acylcarnitines to total carnitine usually is increased.
Cardiovascular dysfunction—A large percentage of symptomatic patients show signs of cardiomyopathies, cardiogenic shock, arrhythmias, and/or sudden death; these conditions are more common in patients who present at a younger age. The exact mechanisms by which these defects lead to the development of cardiovascular manifestations are unknown. As discussed earlier, excess fatty acids may be stored as triglycerides in the cytosol of the myocardial cells. In addition, an inadequate supply of ATP may result in contractile dysfunction and subsequent development of hypertrophic cardiomyopathy. Finally, accumulation of intermediary metabolites, such as long-chain acylcarnitines, may cause myocardial injury and rhythm disturbances.
All disorders of fatty acid oxidation are inherited in an autosomal-recessive manner. Considerable clinical heterogeneity exists within families. The estimated incidence of these defects is 1 in 6000, making these conditions among the more common of the inherited metabolic diseases.
These disorders usually become apparent during the first 2 to 3 years of life. The heart, skeletal muscle, and liver in particular are dependent on mitochondrial oxidation of fatty acids during periods of fasting. Clinical features of these disorders therefore include hypoketotic hypoglycemia, encephalopathy, or Reye-like syndrome associated with increased transaminase concentrations and possibly hyperammonemia, cardiogenic shock, cardiac arrhythmias, and sudden death, particularly during fasting stress. Cardiomyopathy, either dilated or hypertrophic, is present in nearly 50% of patients. Some infants show both ventricular hypertrophy and decreased systolic function. Skeletal lipid-storage myopathy is also seen. The pattern of symptoms in patients who have any specific defect, clinical course, and severity is often unpredictable. Symptoms often appear precipitously. Any situation that increases reliance on fatty acids for generation of ATP may precipitate heart failure or ventricular arrhythmias. In older infants and toddlers, an episode of infection, such as gastroenteritis or pneumonia, may precipitate acute decompensation. In other patients, cardiomyopathy and associated myocardial dysfunction may develop over time. Because these conditions are inherited as autosomal-recessive traits, a family history of sudden death in siblings is relatively common.
An important proportion of patients who have these disorders experience hypoglycemia, hepatic failure, and severe metabolic acidosis within the first 48 to 72 hours after birth. Defects in fatty acid oxidation are estimated to cause about 5% of all cases of sudden infant death. A newborn infant who is breast-fed may be vulnerable until the mother’s milk supply is plentiful. Breast-fed infants may receive little nutrition during the first days of life, and this may result in significant and undue fasting stress, precipitating the acute and sometimes fatal presentation.
More than 20 different specific defects have been described, and those associated with cardiac pathology and/or sudden death in young infants are described as follows (Table 9-5).
Defects in carnitine-dependent transport long- and very long chain fatty acids
Systemic primary carnitine deficiency (plasma membrane carnitine transporter deficiency or carnitine uptake defect) Impaired renal absorption of carnitine is present, and plasma carnitine concentrations are 1% to 5% of normal. About 50% of patients show symptoms in infancy. This defect is often associated with cardiomyopathy, usually dilated, and at times the clinical picture resembles endocardial fibroelastosis. Skeletal muscle weakness may also be present. Acute hepatic encephalopathy superimposed on the cardiomyopathy has been associated with sudden death in infants. Serum carnitine may be normal in the early neonatal period because carnitine is transferred across the placenta. These patients respond very well to carnitine administration and may have complete reversal of cardiomyopathy.
Carnitine-acylcarnitine translocase deficiency This enzyme shuttles acylcarnitines and carnitines between the cytosol and the intramitochondrial matrix space. Most of these patients present in the newborn period with hepatic dysfunction, hypotonia, arrhythmias, and cardiomyopathy, often resulting in sudden death. This is a rare condition with a fairly high mortality. Administration of medium-chain triglycerides, which do not require carnitine for transport into the mitochondria matrix, may be beneficial. The efficacy of carnitine therapy, which is often recommended because of low serum carnitine concentration, is not proven.
Infantile carnitine palmitoyltransferase II (CPTII) deficiency Infants who have <20% of normal enzyme activity present early in life with hypoketotic hypoglycemia, hepatic dysfunction, and cardiovascular collapse associated with hypertrophic cardiomyopathy, often with decreased systolic function and arrhythmias. Life expectancy is very short. Extensive fatty infiltration of the heart, liver, and kidneys is seen at autopsy. These infants usually often have null mutations that result in a complete lack of enzyme activity.
Defects in fatty acid b-oxidation These patients often develop a Reye-like syndrome with associated hyperammonemia, cardiomyopathy, arrhythmias, and sudden death. Serum and urine concentrations of dicarboxylic acids usually are increased.
Very long chain acyl-CoA dehydrogenase (VLCAD) deficiency The majority of patients affected in infancy have cardiac disease. The specific gene defect is heterogeneous and results in variable loss of enzyme activity that is correlated with severity of disease. Patients with markedly decreased enzyme activity present in the newborn period with typical signs and symptoms, and most die within a few months. The cardiomyopathy can be dilated or hypertrophic and may be associated with pericardial effusion and arrhythmias. Long-term survival has been reported in some infants in whom the diet is strictly controlled.
Medium-chain acyl-CoA dehydrogenase (MCAD) deficiency This is one of the most common of the fatty acid oxidation disorders, especially in Caucasians from northern Europe, in whom the predicted incidence (1:6500 to 1:17000) is similar to that of phenylketonuria. This defect is associated with sudden death. Patients do not usually present with symptoms until 3 months of age, but neonatal presentation in association with poor feeding after birth is described. Cardiomyopathy and arrhythmias are less common. Fatty infiltration of the myocardium is seen at autopsy. The prognosis is good with appropriate therapy once the diagnosis is established, especially if the condition is detected by newborn screening before the onset of symptoms.
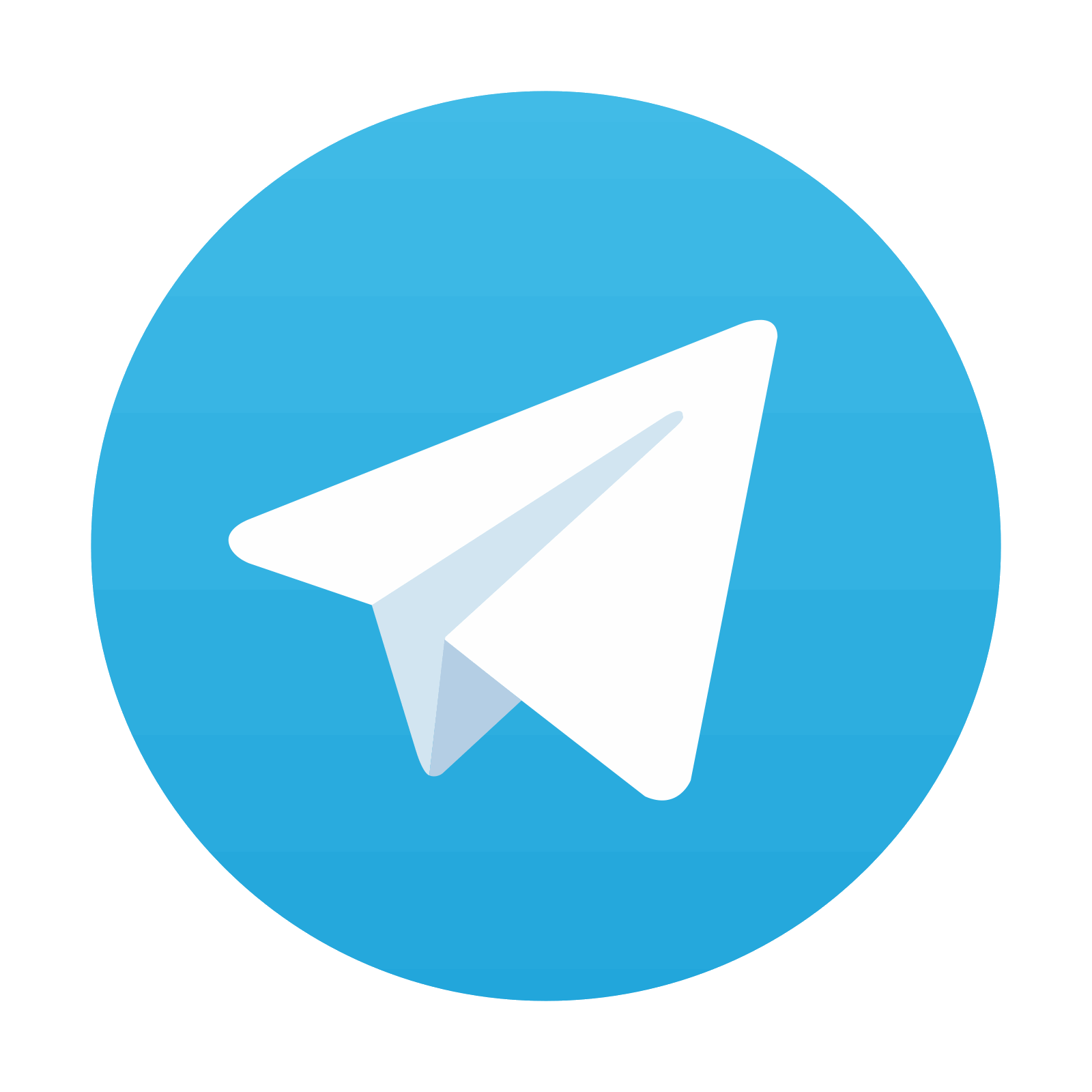
Stay updated, free articles. Join our Telegram channel
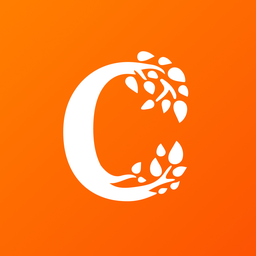
Full access? Get Clinical Tree
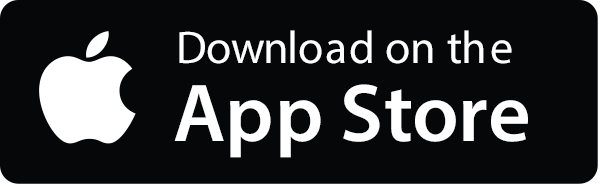
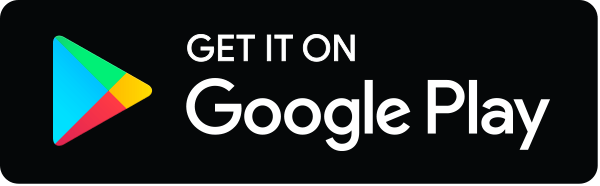