(1)
Project-team INRIA-UPMC-CNRS REO Laboratoire Jacques-Louis Lions, CNRS UMR 7598, Université Pierre et Marie Curie, Place Jussieu 4, 75252 Paris Cedex 05, France
Abstract
Cardiomyocytes (length 70–150μm; width 20–35μm) are striated, nucleated cells that are electrochemically excited to rhythmically contract and relax.
Cardiomyocytes (length 70–150 μm; width 20–35 μm) are striated, nucleated cells that are electrochemically excited to rhythmically contract and relax.
Cardiomyocytes are filled by aligned sarcomeres, i.e., a meshwork of contractile myofibrils enveloped in a network of Ca2 + -storing sarcoplasmic (endoplasmic) reticulum and mitochondria. The sarcolemma, or plasma membrane, invaginate perpendicularly to the cell axis at every Z disc. Transverse tubules reduces the distance between the sarcolemma and the cell axis ( < 1 μm). Transverse tubule surface area represents about 60% of the total cell surface area [328].
In the rat ventricular myocardium, about 1 capillary exists per cardiomyocyte couple for optimized irrigation and oxygenation [329]. However, contracting myocardium creates a pressure field within and outside cardiomyocytes responsible for elevated intramyocardial and intraventricular pressures that affects the lumen of intramural coronary vessels [330].
5.1 External Organization of Cardiomyocytes
Cardiomyocytes are surrounded by a trellis ofcollagen andelastin that supports cells and limits dilation (Fig. 5.1). The helical mesh of collagen prevents excessive cardiomyocyte stretching. Moreover, cardiomyocytes are related to each other by collagen–elastin cross-struts. The 2 sets of fibers develop recoil forces and store mechanical energy during myocardium contraction that can be restored during the followingdiastole [331].
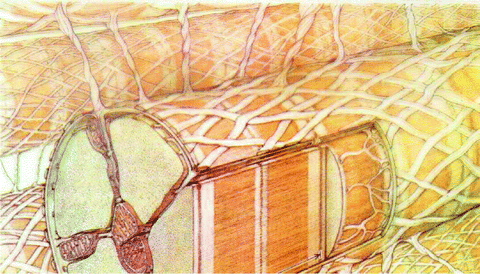
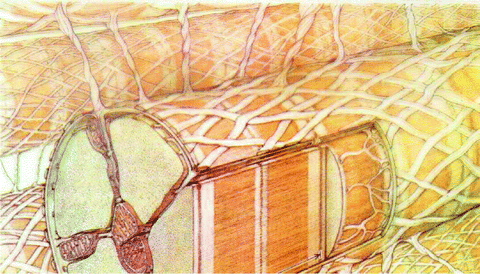
Fig. 5.1
Cardiomyocyte, its collagen envelope and struts; the sarcolemma and its T tubules; inside, the sarcomere, mitochondria and sarcoplasmic reticulum (from [331]).
The collagen network of the left ventricle wall includes 3 major components: (1) a woven collagen network that surrounds groups of cardiomyocytes; (2) array of myocyte-to-myocyte collagen struts (bore 120–150 nm) that extend from the basal lamina of a cardiomyocyte to those of contiguous cardiomyocytes; and (3) array of myocyte-to-capillary collagen struts (of similar caliber) from basal laminae of capillaries to those of contiguous cardiomyocytes [332]. Collagen connections between cardiomyocytes and between them and perfusing capillaries allow bulk motions during the cardiac cycle.
Four types of connective tissues can thus be distinguished: (1) an endomysium- like connective tissue made of beams of collagen fibers that connect cardiomyocytes; (2) connective tissue that links cardiomyocytes and capillaries; (3) perimysium-like connective tissue that forms a sheath of spiral collagen fibers around cardiomyocytes; and (4) epimysium-like connective tissue, i.e., layers of elastin and collagen fibers that limit the endocardic and epicardic surfaces.
5.2 Internal Organization of Cardiomyocytes
Cardiomyocytes contain structural and functional compartments with the nucleus, array of myofibrils, sarcoplasmic reticulum, and mitochondria. These densely packed organelles communicate via functional junctions (e.g., between the sarcoplasmic reticulum and T tubules for electromechanical coupling) and structural contacts (e.g., between the sarcoplasmic reticulum and mitochondria for the control of Ca2 + homeostasis).
5.2.1 Sarcolemma
Cardiomyocyte sarcolemma contains many ion channels, pumps, and exchangers that shape the action potential, the depolarization wave that is received and transmitted to trigger the contraction. Many receptors for extracellular ligands such as neutotransmitters released by the sympathetic and parasympathetic nerves reside in the sarcolemma. Liganded receptors initiate signaling cascades to control the activity of ion carriers. In addition, cardiomyocytes are connected by adhesion sites and gap junctions that propogate electrochemical excitation waves.
5.2.1.1 Intercalated Discs
Cardiomyocytes are joined by regions of interdigitating sarcolemmas, the so-called intercalated discs. They support intercellular communication necessary for proper cardiac function.
These electromechanical junctions contain clusters ofgap junctions to allow electrochemical impulse, oraction potential (APl), to spread rapidly and in a orderly way so the cell contraction is almost synchronized. Cardiomyocytes then act as a syncytium. Stimulation of an individual cell causes the contraction of the entire myocardium.
Intercalated discs contain not only gap junctions for chemical communications, but also mechanical junctions, composed ofadherens junctions (fascia adherens) with N-cadherin, catenins, and vinculin, as well asdesmosomes that contain desmin, desmoplakin, desmocollin, and desmoglein [333].
Adherens junctions and desmosomes dock terminal ends of the myofibrils, intermediate filament network, and microtubules, thereby stabilizing the cytoskeleton and allowing a mechanical coupling between cardiomyocytes. Adherens junctions especially anchor myofibrils and desmin cytoskeleton on cadherin membrane sites. They occupy between-cell interdigitating regions transversely with respect to the sarcomere. On the other hand, desmosomes and gap junctions are located in membrane parts in the direction of the sarcomere axis.
At intercalated discs, zonula occludens protein ZO1 regulates the organization of both gap and adherens junctions [334].Zonula occludens protein ZO1 interacts withCadherin-2 (or N-cadherin); it regulates localization of gap junctions via its association with the cadherin-2 complex.
At intercalated discs,Na
1.5 andK
1.5 channel subunits interact with junctional proteic complexes (intercalated disc interactome) [335].Plakophilin-2, a desmosomal molecule, influences gap junction-mediated coupling as well as Na
1.5 channel function.Cadherin-2 modulates gap junction and K
1.5 functioning. Gap junction proteinconnexin-43 interacts with the sodium channel complex componentankyrin-G, which is required for proper intercellular adhesion and electrical coupling. Connexin-43, which is needed for normal functioning of sodium and potassium channels, regulates ankyrin-G transfer along microtubules into the intercalated disc and conversely.




5.2.1.2 Transverse Tubules
Ventricular excitation–contraction coupling relies on sarcolemmal, transversely oriented, deep, cylindrical invaginations —transverse (T) tubules — that are juxtaposed beside terminal cisternae of the sarcoplasmic reticulum.
Excitation–contraction coupling actually requiresCa2 + ions. Calcium mediator is mainly supplied by thesarcoplasmic reticulum that stores Ca2 + ions fromdiastolic uptake forsystolic release.1
Sarcolemma repeatedly invaginates to form T tubules that plunge into the sarcoplasma (Fig. 5.1). Both sides of T tubules are close to the sarcoplasmic reticulum. In addition, T tubules limit both ends of the sarcomere. Density of T tubules influences Ca2 + influx. Transverse tubules allow to localize sarcolemmal voltage-gated calcium channels and sarcoplasmic reticulum ryanodine receptors2 into close proximity. Cardiac contraction actually results from opening of sarcolemmalvoltage-gated calcium channels that are mostly located in T tubules. These channels trigger Ca2 + influx with local accumulation that activates calcium release from its sarcoplasmic reticulum stores, especially the close junctional regions of this organelle. A decreased number of T tubules, particularly in atrial myocytes and Purkinje cells, is correlated to local slow rate of Ca2 + ingress [337]. The dominant Ca2 + ligand in the sarcomere is troponin-C attached to actin filaments.
Action potential sweeps quickly along the sarcolemma and T tubules to trigger Ca2 + release from the sarcoplasmic reticulum. Owing to its speed, action potential arrives almost simultaneously at all tubules of the T-tubule network to ensure that all sarcomeres have a coordinated contraction. Calcium ions then diffuse to bind to troponin.3 This bond leads to the interaction between actin and myosin and sarcomere contraction. When the process ends, Ca2 + is pumped back into the sarcoplasmic reticulum (Sect. 6.6).
The formation and maintenance of T tubules require myotubular myopathy proteinMTM1, or myotubularin-1 [338]. This phosphoinositide 3-phosphatase acts on both phosphatidylinositol 3-phosphate and (3,5)-bisphosphate. Myotubularin activity on PI(3)P and/or PI(3,5)P2 may interfere with channel activaty.
On the other hand, atriomyocytes of many mammalian species do not express extensive T-tubule networks. Coupling of Ca
channels and ryanodine receptors occurs at dyadic junctions at the cell periphery. Peripheral Ca2 + ions do not diffuse into the center of atriomyocytes. Three-dimensional non-junctional lattice of ryanodine receptors may sense cortical Ca2 + signals and transmit them to the cell center via a Ca2 + release from the sarcoplamic reticulum. This lattice becomes active and allows a centipetal diffusion of Ca2 + ions when a greater atrial contraction is required.

5.2.1.3 Plasmalemmal Nanodomains
Membrane rafts andcaveolae (50–100 nm plasmalemmal invaginations) constitute dynamic platforms that recruit ion channels and their partners to build channelosomes. Certain cardiac channel subunits that shape the cardiac action potential (Sect. 5.10) actually localize to membrane rafts and/or caveolae, such as those of Ca
1.2, K
K
6.2), K
2, K
3, K
1.4, K
1.5, K
2.1, and Na
1.5 (Table 5.1) [339]. Ion channel partners, receptors of nervous control, and protein kinases and phosphatases that coreside in channelosomes modulate localization and/or activity of ion channels.









Table 5.1
Ion channels in caveolae and membrane rafts of the sarcolemma of nodal cells and cardiomyocytes (Source: [339]). Among hyperpolarization-activated and cyclic nucleotide-gated (HCN) channels, HCN4 is the main isoform in the sinoatrial node; HCN1 and HCN2 are also synthesized.
Channel | Membrane localization |
---|---|
Ca ![]() | Caveolae |
IP3R | Caveolae |
KV1.4 | Membrane raft |
KV1.5 | Caveolae (ventriculomyocyte) |
Membrane raft (atriomyocyte) | |
KV2.1 | Membrane raft |
KV4.2 | Membrane raft |
KIR2.1 | Membrane raft |
KIR3.1 | Membrane raft |
KIR6.1 | Membrane raft |
KIR6.2 | Membrane raft |
KCa | Membrane raft |
NaV1.5 | Caveolae (ventriculomyocyte) |
HCN4 | Caveolae (nodal cell of sinusal node) |
Caveolin-3 associates withdystrophin andβ-dystroglycan that are components of thedystrophin-associated protein complex. Dystrophin andα-actinin anchor caveolae to the actin cytoskeleton [339]. Sodium channels that localize in cardiomyocyte T-tubular caveolae interact specifically withsyntrophin, another constituent of the DAPC complex [339].
The cAMP-dependent regulation of voltage-gated calcium channels CaV1.2 relies on interaction betweenA-kinase anchoring proteins andprotein kinase-A. A-kinase anchoring proteins recruit protein kinase-A near ion channels in membrane rafts and caveolae. Channel CaV1.2 assembles with β2-adrenoceptor, Gs subunit of G protein,adenylate cyclase, AKAP, PKA, phosphatasePP2, and caveolin-3 [339]. Protein kinase-A as well ascalmodulin-dependent protein kinase CamK2 phosphorylate NaV1.5 and CaV1.2 channels, ryanodine-sensitive Ca2 + -release channel RyR2,4 and phospholamban regulator of sarco(endo)plamic reticulum calcium ATPase.
Disc large homolog DLg4 is required for KV1.4 localization in membrane rafts [339]. In the atrial myocardium, KV1.5 channels lodge inintercalated discs that lack caveolin-3. Disc large homolog DLg1 that binds tocadherin promotes KV1.5 localization in membrane rafts. In intercalated discs,connexin-43 resides in membrane rafts, where it links to N-cadherin.
Channel KIR2 complexes with DLg1 and DLg4 adaptors [339]. In membrane rafts, DLg1 and DLg4 tether other scaffold proteins, such as calcium–calmodulin-dependent protein Ser kinase (CASK) of the membrane-associated guanylate kinase (MAGUK) family, vertebrate Lin7 homolog VeLi1, and Munc18-1-interacting protein MInt15 to form a complex that recruits KIR2 to the plasma membrane [339].
Both DLg1 and DLg4 connect also toCa2 + -activated calmodulin-dependent kinase CamK2 that regulates numerous ion channels according to intracellular Ca2 + concentration. Most channels regulated by CamK2 localize in membrane rafts and caveolae with CamK2, such as CaV1.2, KV1.5, IP3R, and Na + channels [339].
In addition, DLg1 and DLg4 link to A-kinase anchoring proteins. Channel KV7.1 associates with both PKA and PP1phosphatase via AKAP9 [339]. The AKAP proteins interact also with β2-adrenoceptors. Therefore, channelosomes can be controlled by the sympathetic system.
Like β-adrenergic receptor and its G protein, adenylate cyclase, and protein kinase-A, α1 adrenergic receptor, caveolin-3, Gq subunit of G protein, andphospholipase Cβ subtypes (PLCβ1 and PLCβ3) are confined to caveolae [340]. A decrease in caveolin-3 density may contribute to the development of cardiac maladaptivehypertrophy.
5.2.2 Sarcoplasmic Reticulum
The sarcoplasmic reticulum is a smooth endoplasmic reticulum in myocytes. Whereas the endoplasmic reticulum synthesizes molecules, the sarcoplasmic reticulum stores, pumps, and expelscalcium ions. The sarcoplasmic reticulum sequesters a large amount of calcium that can be released on the arrival of the action potential owing to special connections between the sarcoplasmic reticulum and the sarcolemma with T-tubules.
The sarcoplasmic reticulum located throughout the myoplasm can be divided into 3 main components: junctional (JSR), corbular (CSR), and network (NSR). The sarcoplasmic reticulum indeed widens at multiple sites to form junctional SR cisternae tightly coupled to the sarcolemma of T tubules. The junctional compartment is positioned within 12 to 15 nm to T tubules. Together with the T tubule, JSR defines a restricted subspace in which Ca2 + can reach high concentration. Junctional sarcoplasmic reticulum contains the majority of theryanodine receptors.
Calcium release from sarcoplasmic reticulum storage occurs at discrete loci in the JSR–T tubule subspace —Ca2 + sparks, although other secondary Ca2 + transient currents are initiated by other ion carriers. The corbular region is located near the T tubules at a distance of several micrometers from the sarcolemma. Corbular sarcoplasmic reticulum releases Ca2 + without interaction with sarcolemmal CaV1.2 channels. Sarcoplasmic reticulum Ca2 + ATPases are predominantly located in network sarcoplasmic reticulum.
In cardiomyocytes, the cardiac Ca2 + release unit is a JSR proteic complex that comprises CaV1.2 juxtaposed to ryanodine-sensitive, Ca2 + -release channel RyR2, thereby achieving excitation–contraction coupling. This coupling is controlled by RyR2-associated proteins and RyR2 phosphorylation. Junctional sarcoplasmic reticulum unit includesryanodin receptor RyR2,calsequestrin Casq2,junctin, junctophilin-1 and -2, andtriadin. Triadin-1 is the predominant isoform in cardiomyocytes. The JSR complex is involved in Ca2 + release from the sarcoplasmic reticulum by Ca2 + release units to trigger myocyte contraction, as well as buffering of stored Ca2 + in the lumen of the sarcoplasmic reticulum. In addition, Ca2 + -induced Ca2 + release units exert anegative feedback on CaV1.2 channels. Conversely, muscle relaxation results from Ca2 + reuptake into the sarcoplasmic reticulum by sarco(endo)plasmic reticulum Ca2 + ATPases.
Calsequestrin-2 sequesters Ca2 + inside the sarcoplasmic reticulum lumen, avoiding Ca2 + leaks from its store during myocyte relaxation. Junctin docks Casq2 to RyR2. Junctophilin-1 and -2 promote colocalization of CaV1.2 with RyR2. In addition, Aspartyl–asparaginyl β-hydroxylase (AspH) participates in calcium storage in and release from the sarcoplasmic reticulum. Triadin anchors calsequestrin Casq2 to RyR2 ryanodine receptors. Triadin maintains the structural and functional integrity of the cardiac Ca2 + release units of the sarcoplasmic reticulum [341].
Ca2 + -binding calmodulin andCa2 + –calmodulin-dependent CamK2 kinase regulate activity of ryanodine receptors and phospholamban. Calmodulin mediates Ca2 + -dependent channel inactivation.
Calcium-induced calcium release from the sarcoplasmic reticulum through ryanodine receptors initiated by Ca2 + influx through CaV channels is an amplification process relying on a positive feedback mechanism that governs cardiac inotropy. Each cardiomyocyte produces a Ca2 + transient. The amplitude of Ca2 + transients is determined by recruitment level of Ca2 + sparks (elementary Ca2 + sarcoplasmic reticulum release signals), i.e., by increasing the number of RyR channels clustered in spark-forming couplons. Sensitization and inhibition of RyR clusters shorten and lengthen the interval between consecutive Ca2 + n sparks, respectively [342]. Cytosolic calcium concentration rises and falls very rapidly upon opening and closing of RyR channels to ensure adequate sarcoplasmic reticulum emptying and refilling through SERCA pumps, hence properinotropy andlusitropy.
Calcium spark termination may be induced by junctional Ca2 + concentration within the sarcoplasmic reticulum lumen that enables RyR desensitization and inactivation, once a given threshold is reached [342]. Increased or decreased buffering capacity of the sarcoplasmic reticulum prolongs or abbreviates the duration of Ca2 + release, respectively. Sensitization of RyRs with caffeine or inhibition of RyRs with tetracaine heightens or diminishes the rate of triggering probability recovery, respectively [342]. Sensitivity of RyR channels does not influence Ca2 + spark amplitude recovery; the latter relies on local sarcoplasmic reticulum refilling. On the other hand, Ca2 + spark triggering depends on both refilling and RyR sensitivity.
Calcium release refractoriness, which reduce the probability of channel reopening during a given time interval after closure, permit to avoid arrhythmogenesis. Calcium release refractoriness can relies, at least partly, on the rate-limiting conformational change of a Ca2 + flux controller such as calsequestrin. Recovery of Ca2 + release through RyRs depends on the CaV channel trigger, between-beat changes in sarcoplasmic reticulum SR Ca2 + content, and β-adrenoceptor acitivity that controls cardiacfrequency [342].
5.2.3 Mitochondria
Cardiomyocytes have a richer supply ofmitochondria and greater dependence onadenosine triphosphate (ATP) than skeletal myocytes. Mitochondria cluster in the vicinity of the nucleus and enter into contact with it to support energy requirement for nuclear function. Mitochondria are also close to the sarcoplasmic reticulum and sarcomere to minimize molecular transfer.
5.2.3.1 Mitochondria Populations
In the cardiomyocyte, 2 functionally and biochemically distinct populations of mitochondria exist [343]: (1) subsarcolemmal mitochondria that are located beneath the sarcolemma and (2) interfibrillar mitochondria that are situated among myofibrils. Activities of succinate dehydrogenase and citrate synthase are higher in interfibrillar mitochondria with respect to subsarcolemmal mitochondria, whereas those of carnitine palmitoyltransferase and α-glycerophosphate dehydrogenase are nearly the same in both mitochondrium types. Interfibrillar mitochondria oxidize tested substrates with a rate about 1.5 times faster than that of subsarcolemmal mitochondria.
5.2.3.2 Electron Transport Chain
Theelectron transport chain, composed of 4 main proteic complexes (Table 5.2) in the mitochondrion inner membrane, enablesoxidative phosphorylation. Reducednicotinamide adenine dinucleotide (NADH)6 and succinate generated in thecitric acid cycle, also called tricarboxylic acid and Krebs cycle, are oxidized to release protons. In redox reactions, electrons are transferred from electron donors such as NADH to electron acceptors such as oxygen. Redox reactions release energy that powers ATP synthase. Electrons carried through the electron transport chain are used to transport protons across the inner mitochondrial membrane. This process creates a potential energy in the form of a proton concentration (pH) gradient and electrical potential across the inner mitochondrial membrane. Protons move back across the membrane and down their concentration gradient through ATP synthase that uses stored energy. Within the inner mitochondrial membrane, the lipid-soluble electron carrier coenzyme-Q10 carries both electrons and protons from its oxidized ubiquinone form (UQ; a.k.a. quinone Q) to its reduced ubiquinol form (UQH2 or QH2). In the coenzyme-Q10 redox cycle, oxidized ubiquinone UQ accepts 2 electrons and 2 protons, whereas reduced ubiquinol UQH2 releases 2 electrons and 2 protons.
Table 5.2
Electron transport chain and ATP synthase (CytC: cytochrome-C; IMS: inner mitochondrial membrane; MM: mitochondrial matrix; O2 − : superoxide anion; P i : inorganic phosphate). The inner mitochondrial membrane is impermeable to most metabolites and ions, thereby creating a transmembrane potential and ionic gradient. Reactive oxygen species are formed at complex-I and -III of the respiratory chain. Superoxide anion produced from the transfer of an electron from the partially reduced ubiquinone to oxygen is then reduced to hydrogen peroxide (H2O2) by superoxide dismutase. Hydrogen peroxide is eliminated by glutathione peroxidase or reacts with ferrous ions and other transition metals to form hydroxyl radical.
Complex | Alias |
---|---|
Reaction | |
I | NADH–coenzyme-Q oxidoreductase, NADH dehydrogenase |
NADH + UQ + 5H ![]() ![]() ![]() | |
O2 + e ![]() | |
II | Succinate–coenzyme-Q oxidoreductase |
(made of heme, iron–sulfur clusters, and | |
flavin adenine dinucleotide) | |
Succinate + UQ ![]() | |
III | Coenzyme-Q–Cytochrome-C oxidoreductase, cytochrome-BC1 complex, |
cytochrome-C reductase | |
(made of iron–sulfur cluster, cytochrome-C1, and 2 cytochrome-B) | |
UQH2 + 2 CytC ox + 2H ![]() | |
O2 + e ![]() | |
IV | Cytochrome-C oxidase |
4 CytC red + O2 + 8H ![]() ![]() | |
V | ATP synthase, F1F0 ATPase |
(F O proton channel and F1 synthase domain) | |
ADP + P i + 4 H ![]() ![]() |
The electron transport chain is composed of 4 major complexes: NADH–ubiquinone reductase (complex-I), succinate–ubiquinone reductase (complex-II), ubiquinol–cytochrome-C reductase (complex-III), and cytochrome-C oxidase (complex-IV). Electron transfer between these complexes is ensured by interactions between electron carriers coenzyme-Q10 and cytochrome-C.
Cardiolipin can link complex-III and -IV. In addition, complex-I is stabilized by binding to complex-III in human mitochondria. Like complex-I, -III, and -IV (but not complex-II), ATP synthase (complex-V) is able to form supercomplexes by oligomerization [344]. A dimeric state of ATP synthase is specific for mitochondria.Respirasomes refers to respiratory chain supercomplexes such as the aggregate made by complex-I, -III dimer, and -IV (respirasome-I–III–IV). These supercomplexes reduce electron diffusion distance.
5.2.3.3 PGC1α andPGC1β
Peroxisome proliferator-activated receptor-γ (or nuclear receptor NR1c3) coactivatorsPGC1α and PGC1β are highly expressed in the heart. The expression of PGC1α rises whenATP production must increase, i.e., when the cardiac activity increases. Regulator PGC1α coactivates PPARα (nuclear receptor NR1c1), estrogen-related receptor ERRα (NR3b1), and other nuclear factors that control the transcription of genes involved in cardiac fatty acid oxidation and mitochondrial functional capacity [345].
Moreover, PGC1α stimulates the mitochondrial genesis in cardiomyocytes, although it is not essential for mitochondrial generation. In the absence of PGC1α, mitochondrial density remains normal, but expression of the genes of oxidative phosphorylation decays [346]. Homeostasis of ATP and phosphocreatine is disturbed. The Gs-coupled βAR–cAMP pathway,calmodulin-binding catalytic subunit of PP3,calcium–calmodulin-dependent protein kinase,AMP-activated protein kinase,P38MAPK, andnitric oxide activates PGC1α expression.
5.2.3.4 Mitochondrial Permeability Transition Pore
Mitochondrial permeability transition pore (mPTP) is a non-selective, large- conductance channel that remains normally closed. It is partly constituted of: (1) a dimer ofvoltage-dependent anion channels (VDAC),7 or porins, in the mitochondrial outer membrane (MOM); and (2) a dimer ofadenine nucleotide translocases (ANT)8 linked to (3) mitochondrial inorganic phosphate (Pi) carrier SLC25a39 in the mitochondrial inner membrane (MIM) [347]. Both VDAC and ANT serve as regulators of the mPTP in cooperation with the matrix protein,cyclophilin-D (CyPd).10
Adenine nucleotide translocase interacts with cyclophilin-D and SLC25a3 carrier. Binding of cyclophilin-D to mPTP increases mPTP sensitivity to Ca2 + ion. Elevation of matrix Ca2 + concentration triggers mPTP opening and cytosolic-open ANT conformation, thereby favoring Ca2 + sensitivity of the mitochondrial permeability transition pore.
Opening of mitochondrial permeability transition pore causes mitochondrial swelling, hence rupture of the mitochondrial outer membrane and release of cytochrome-C into the cytosol, triggering apoptosome formation and myocyte death. Multiple prosurvival signals prevents mPTP opening (Table 5.3). On the other hand, mPTP remains closed during ischemia and opens shortly after reperfusion. Moreover, ischemia–reperfusion events promote CyPd–PiC–ANT interaction in cardiomyocytes.
Table 5.3
Prosurvival signaling pathways triggered by G-protein-coupled, protein Tyr kinase, and cytokine receptors that target mitochondria (Source: [347]; GSK: glycogen synthase kinase; HK: hexokinase; JaK: Janus kinase; mKATP: mitochondrial ATP-sensitive K + channel; PKC: protein kinase C; ROS: reactive oxygen species; STAT: signal transducer and activator of transcription;
: inhibition). Certain mitochondrial protein kinases in cardiomyocyte ensure protection against ischemia–reperfusion damage. On the other hand, GSK3β phosphorylates porin, thereby releasing HK2 from mitochondria and provoking an oxidative stress, in addition to activation of mitochondrial permeability transition pore. On the other hand, PKCε phosphorylates porin, but HK2 remains attached to porin. Kinase PKA phosphorylates also porin, thereby supressing its opening.

Cytosol | Mitochondrion |
---|---|
JaK–STAT3 | STAT3 (mPTP ![]() |
MAP2K1/2–ERK1/2–NOS3–PKG | PKCε–PKB–HK2 (mPTP ![]() |
PI3K–PKB–ERK1/2–NOS3–PKG | PKCε–PKB–GSK3β (GSK3β ![]() |
PKCε–PKB–ERK1/2–NOS3–PKG | PKCε–mK ![]() |
-ROS–ERK1/2–GSK3β (GSK3β ![]() | |
-ROS–PKCε–GSK3β (GSK3β ![]() | |
-ROS–PKCε–AldH2 |
The mPTP regulation depends onhexokinase-2,11glycogen synthase kinase-3β,12signal transducer and activator of transcription STAT3,13 andsirtuin-3 [347].14
Brief periods of ischemia and reperfusion, the so-called ischemicpreconditioning, protect the myocardium against injury by a subsequent sustained ischemia. Binding of HK2 to mitochondria rises after ischemic preconditioning and insulin stimulation that activate PI3K–PKB axis. Deacetylation of cyclophilin-D (Lys166) by sirtuin-3 reduces its PPIase activity [347]. Cardioprotection is also ensured by STAT3 phosphorylation (Tyr705) [347]. Factor STAT3 can attenuate ischemia-induced ROS production in cardiomyocytes.
In cardiomyocytes, GSK3β is predominantly located in the cytosol. Ischemia–reperfusion events cause GSK3β translocation from the cytosol to mitochondria. Cardioprotection by mitochondrial ATP-sensitive K + channel before ischemia relies on mitochondrial GSK3β phosphorylation (Ser9; inactivation) [347].
In ventriculomyocytes, connexin-43 phosphorylation at Ser or Tyr residues regulates gap junction permeability, hence electrochemical coupling of cardiomyocytes. Connexin-43 also localizes to the inner membrane of subsarcolemmal mitochondria. It increases mK
channel activity, leading to elevated ROS production.

Glycogen synthase kinase-3β operates via mitochondrial inner membraneconnexin-4315 and mitochondrial inner membraneATP-sensitive K + channel [348]. cardioprotection relies on opening of mKATP channel. ActivatedPKC kinase and inhibitedPP2 phosphatase increase the open probability of mKATP channels via GSK3β and mitochondrial connexin-43 hemichannels, which can carry ATP. Phosphorylation of mitochondrial connexin-43 increases its activity. Ischemic preconditioning is supported by phosphorylation (inhibition) of glycogen synthase kinase-3β, thereby repressing mitochondrial permeability transition pore opening.
In cardiomyocytes, connexin-43, together with Gβ subunit of G protein, participates in PI3K
activation, hence in the cytoprotective PI3K–PKB–GSK3β pathway [349].16 In the myocardium, the PI3K–PKB–GSK3β axis enables ischemia tolerance supported by insulin, erythropoietin, bradykinin, and adenosine receptors. The PI3K–PKB pathway stimulates ROS production by opening of mitochondrial ATP-sensitive K + channel that requires a certain mitochondrial concentration of connexin-43.Endothelin-1 that acts via G-protein-coupled receptors and signals in cooperation with connexin-43, andinsulin-like growth factor-1 that targets receptor protein Tyr kinase, without the assistance of connexin-43, cause phosphorylation of PKB and GSK3β. In other words, connexin-43 is involved in cell protection ensured by endothelin-1, but not that primed by IGF1 agent. Endothelin-1 can elicitROS production in mitochondria using connexin-43, but not IGF1 factor. Connexin-43 is involved in the activation of the PKB–GSK3β axis by PI3K
possibly via its interaction with the Gβ subunit of G protein, but not via mitochondrial ROS production.


5.2.3.5 Myoglobin
Myoglobin is an iron- and oxygen-binding protein of myocytes that functions in oxygen transport and storage. In addition, as a reductase, deoxygenated myoglobin generatesnitric oxide from circulating nitrite. On the other hand, oxymyoglobin acts as a dioxygenase to convert NO into nitrates [350]. Low concentration of nitrite ions (NO2 − ) cause vasodilation and suppression of mitochondrial activity, thereby reducing generation of reactive oxygen species, especially after ischemia–reperfusion events. Owing to nitrite reductase, nitrite ions generate nitric oxide, a vasorelaxant that decreases cardiomyocyte contractility andcardiac frequency by repressing the electron transport chain in mitochondria.
5.2.3.6 Mechanotransduction
Mitochondrial depolarization and K + accumulation in the mitochondrial matrix by activatedATP- orCa2 + -dependent K + channels and inhibitedH + –K + exchanger strongly increase contractile force in rat ventriculomyocytes [351]. Change in the mitochondrial ionic balance causes mitochondrial swelling that can compress the myofibrillar and nuclear compartments of cardiomyocytes that are sensitive to extracellular mechanical stimuli. A relatively small increase in mitochondrial volume can cause a relatively large reduction in sarcomeric lattice spacing in the absence of strong stretch of cardiomyocytes and concomitant increase in passive force [351].
When myosin and actin filaments are closer, both myofilament Ca2 + sensitivity and maximal Ca2 + -induced tension increase. In addition, mechanical stresses applied on the nucleus can influence both the DNA accessibility for gene transcription and the nucleocytoplasmic transport of molecules. Therefore, generated internal pressure that affects morphological and functional properties of organelles contributes to theregulation of cell functioning [351].
5.2.3.7 Cardioprotection
Cardioprotection relies on [352]: (1) activation of numerous cardioprotective kinases, such asextracellular signal-regulated protein kinase,phosphatidylinositol 3-kinase, andprotein kinase B; (2) translocation to the mitochondria of kinases (e.g., PKB, PKCε,17glycogen synthase kinase GSK3, glyceraldehyde 3-phosphate dehydrogenase, and hexokinase, among others), phosphatases, and other signaling proteins;18 (3) post-translational modifications of mitochondrial proteins, such as phosphorylation, S-nitrosylation,19 acetylation, Olinked βNacetylglucosamine glycosylation, glutathiolation, sumoylation, and ubiquitination; and (4) inhibition of themitochondrial permeability transition pore.
The mitochondrial permeability transition pore comprises outer membrane voltage-dependent anion channel, inner membrane, regulatory adenine nucleotide transporter, and matrix cyclophilin-D, in addition to mitochondrial phosphate carrier.
Underhypoxia, mitochondria generate toxicreactive oxygen species. The electron transport chain is the main ROS source. Nevertheless, cardiomyocytes subjected to hypoxia keep mitochondrial activity under control, as they are equipped with oxygen sensors prolyl hydroxylases and hypoxia-inducible factors [353].
During hypoxia, increased glycolysis causes a lactic acid accumulation, hence intracellular pH reduction. Consequently,Na + –H + antiporter is activated and raises intracellular Na + concentration that cannot be corrected byNa + –K + ATPase due to the decline in ATP concentration. Increased intracellular Na + concentration leads to elevated intracellular Ca2 + concentration becauseNa + –Ca2 + exchanger is inhibited or reversed [353].
Reactive oxygen species are formed at complex-I and -III of the electron transport chain and mainly via xanthine oxidase on xanthine formed by adenosine degradation. Reactive oxygen species can inhibit ATP synthase and adenine nucleotide translocase. They can also cause peroxidation of unsaturated fatty acids in membrane phospholipids such as cardiolipin at the inner mitochondrial membrane that then prevents respiratory chain activity. The combined effects of ROS and augmented Ca2 + n concentration in the mitochondrial matrix causes opening of the non-specificmitochondrial permeability transition pore, hence free passage of small cytosolic molecules ( < 1.5 kDa). Therefore, pore opening increases the mitochondrial matrix volume, disrupts mitochondrial outer membrane, and provokes cell death (Vol. 2 – Chap. 4. Cell Survival and Death).
Ischemic postconditioning that consists of alternating, brief periods of ischemia and reperfusion is effective when applied at the onset of reperfusion. It includes formation and release of autacoids and cytokines, maintenance of acidosis during early reperfusion, activation of protein kinases, and repression of opening of mitochondrial permeability transition pore.
During hypoxia, as HIFα is not targeted by the oxygen sensor prolyl hydroxylase that enables it interact withvon Hippel-Lindau ubiquitin ligase for proteasome degradation, this subunit accumulates and forms HIFαβ heterodimer.20 Following the recruitment of CBP and P300 coactivators, HIF causes target gene transcription. Hypoxia-inducible factor provokes [353]: (1) expression of glucose transporters GLUT1 and GLUT4; (2) that of glycolytic enzymes to balance decayed cell respiration; (3) that of lactate dehydrogenase-A that converts pyruvate into lactate, hence diverting pyruvate away from the mitochondria; (4) that of pyruvate dehydrogenase kinase that impedes conversion of pyruvate into acetyl coenzyme-A, which enter the Krebs cycle; (5) switch form COx4-1 to COx4-2 subunit in complex IVto optimize the efficiency of electron transport; and (6) mitochondrial autophagy. In addition, to prevent mitochondrial permeability transition pore opening, HIF1α elicits [353]: (1) adenosine production to stimulate the PI3K–PKB pathway; (2) NO generation by NOS2 to activateprotein kinase-G for opening of mitochondrial KATP channels that promotes production of low levels of ROS messengers; as well as (3) activation of inner mitochondrial membrane uncoupling proteins UCP2 and UCP3 to limit mitochondrial generation of ROS concentrations.Produced ROS messengers oxidize (inactivate)PTen, thus activating survival PKB kinase.
5.2.4 Nuclear GPCRs
G-protein-coupled receptors, such as α1- and β-adrenergic, angiotensin-2 AT1 and AT2, apelin, bradykinin B2, endothelin, metabotropic glutamate, lysophosphatidic acid, platelet-activating factor, and prostaglandin receptors target the nuclear membrane [354].
Furthermore, numerous GPCRs contain nuclear localization sequences that enable them to cross nuclear pore complexes, hence to move from the outer to the inner nuclear membrane, thereby delivering signals toward the cytoplasm or the nucleoplasm.
Heterotrimeric G proteins transmit stimuli from plasmalemmal GPCRs to various intracellular effectors, such as enzymes and ion channels. Associated signaling mediators, such as heterotrimeric G proteins, adenylate cyclases, phospholipase-A2, -Cβ, and -D, regulators of G-protein signaling, β-arrestin-1, GPCR kinases, A-kinase-anchoring proteins, and protein kinase-A, among others, can be transferred to the nuclear membrane and nucleus. Moreover, enzymes involved in the synthesis and metabolism of phosphoinositides as well as in the processing of peptide ligands, such as angiotensin-converting enzyme and endothelin-converting enzyme-1 can localize to the nucleus.
Both plasmalemmal and nuclear GPCRs can activate effectors, such as G proteins, β-arrestins, and nuclear protein kinases that modulate the activity of transcription factors. Gβγ subunits as well as effectors PKB and MAPK interact with transcription factors, chromatin regulators such as histone deacetylases, and DNA elements [354].
In addition, Gβγ subunits regulate cellular signaling and organize the assembly and transfer of receptor-based complexes in intracellular compartments, such as the endoplasmic reticulum and Golgi body. Moreover, Gβ1γ2 dimer can localize in the nucleus, where it interacts directly withHDAC5 histone deacetylase [354]. The Gβ5–RGS7 complex localizes to both the cytosol and nucleus. Protein RGS6 can also reside in the nucleus. In the nucleus, Gβγ5 dimer complexes with the transcriptional repressor adipocyte enhancer-binding protein AEBP1 and attenuates its transcriptional repression. In addition, Gβγ colocalizes with activating protein AP1 in the nucleus and recruits HDACs to inhibit AP1 transcriptional activity. Prenylation increases Gβγ amount associated with the glucocorticoid receptor (NR3c1) in the nucleus.
Nuclear GPCRs launch classical pathways that produce and/or activate a second messengers or stimulate the MAPK module or the PI3K–PKB axis, like those initiated at the plasma membrane. Nonetheless, these receptors can directly regulate DNA synthesis, histone modification, and gene transcription [354].
5.2.4.1 Nuclear β-AdrenergicReceptors
In cardiomyocytes, plasmalemmalβ1-adrenergic receptors launch the Gs–AC–cAMP pathway responsible for thechronotropic and inotropic effects of sympathetic stimulation. β-Adrenergic receptors can also interact with other heterotrimeric G-protein subunits such as Gi subunit. Other signaling pathways rely G-protein-coupled receptor kinases and β-arrestins. β-Arrestins are implicated in GPCR desensitization, hence suppression of the first wave of signals triggered at the plasma membrane by G proteins, and internalization, which is associated with an (eventually G-protein-independent) intracellular (endosomal) second wave of cues.
In cardiomyocytes, whereas plasmalemmal β1-adrenergic receptors play a predominant role in the regulation of cardiomyocyte contractility, plasmalemmal β2-adrenergic receptors play a more modest role in regulation of cardiacinotropy andlusitropy.
β-adrenoceptors can colocalize with NuP62 nucleoporin. In fact, Gs-coupled-β1- and Gi-coupled-β3-adrenoceptors, but not β2-adrenoceptors, can lodge in the nuclear membrane, at least in rodent adult ventriculocardiomyocytes [354]. Whereas β1AR activates adenylate cyclases, β3AR modulates transcriptional initiation. Stimulated βARs target rRNA and mRNAs, such as transcripts of NFκB and components of its signaling pathways. Extracellular noradrenaline is taken up and can move to the nucleus within hours.
5.3 Sarcomere
Myofibrils, formed by packed contractile proteins (actin, myosin, and associated proteins), fill a large fraction of the cytosol, whereas the rest is occupied by other organelles such as mitochondria.
The striated appearance of myofibers is created by a transverse pattern of alternating dark A bands,21 bisected by the H zone22 with a mid M line,23 and light I bands,24 divided by Z lines, or Z discs,25 (Fig. 5.2).
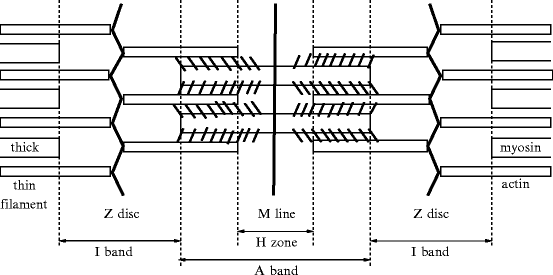
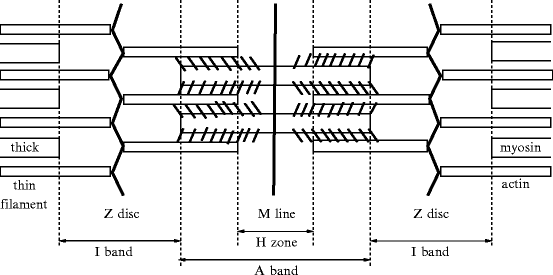
Fig. 5.2
The sarcomere, its microscopic structure and the myofilament arrangement. The M line and the Z discs are proteic scaffolds that orientate myosin and actin filaments, respectively. The H zone (bisected by M line) contains only thick myosin filaments, the I band (bisected by Z disc) only thin actin filaments, and the region of the A band close to the Z disc both filament types.
The sarcomere (length ∼ 2 μm), the array of thick and thin filaments between the Z lines, is the anatomical unit of muscular contraction, and the hemisarcomere is the functional unit. Repeating sarcomere units are precisely aligned in the cardiomyocyte.
The sarcomere length is a predictor of muscle function. Non-invasive measurements have been developed to assess the sarcomere structure. Tissue scattering is related to refractive index distributions associated with the morphology and composition of the explored tissue. Sarcomere structural changes can be monitored by optical reflectance on muscle, a strong correlation existing between muscle optical scattering properties and sarcomere length used as a predictor of muscle function [355]. Visualization of individual sarcomeres and analysis of their dynamics can now be obtained owing to optical microendoscopy [356].26
The sarcomere includes 4 filament complexes: (1) thin filament that is predominantly made of
actin,27 (2) thick filament, mainly consisting of myosin28 (3) titin,29 and (4) nebulin. The lateral boundaries of the sarcomeric units, Z discs, relate contractile apparatus to cytoskeleton. Sarcomere shortening of striated myocyte results from actin and myosin filaments sliding over each other, as the lengths of these filaments remain constant.

Sarcomere Z discs that anchor myofilaments and stabilize their assembly, are densely packed cellular structures that include actin, titin, and nebulin, as well as smaller proteins, such as α-actinin andtelethonin. Two giant titins are assembled into a complex by telethonin, a Z-disc ligand that has a symmetric structure [358].
The molecular chaperone, heat shock protein HSPb5,30 abounds in the myocardium. It may act in the assembly and remodeling of sarcomeres. Under normal conditions, HSPb5 lodges in the cytosol; it is only slightly linked to the cytoskeleton. During stress, it moves to the Z disc of the sarcomere and can interact with actin, myosin, desmin, titin and vimentin, hence stabilizing sarcomeric and cytoskeletal structures. Once phosphorylated by P38MAPK and MAPKAPK2 and then translocated to mitochondria, it protects against acute metabolic stresses, such as ischemia and reperfusion, as it also maintains mitochondrial integrity, hence impeding H2O2-induced cytochrome-C release [360]. Chaperones HSPb5 and HSP47 might play complementary roles in cross-bridging actin filaments in the Z disc of the sarcomere.
5.3.1 Myofibrils
Each myofibril is made of arrays of parallel filaments. In the H zone, the thick and thin filaments never overlap (Figs. 5.3).
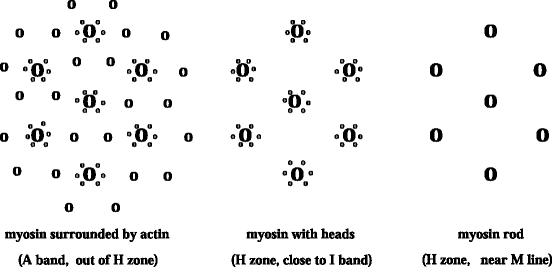
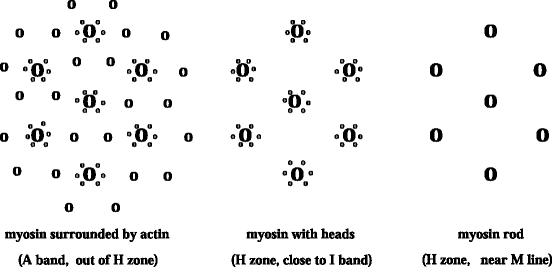
Fig. 5.3
Sarcomere cross-section and myofilament arrangement. The inner region of the H zone (in the M line and its vincinity) contains the rod part of the myosin filament (bare zone). Myosin heads are located in the outer regions of the H zone and A band. Myosin filaments are surrounded by actin filaments in the outer region of the A band. The I band (not represented) is composed only by actin filaments.
5.3.2 Myosin Thick Filaments
The thick filament (diameter ∼ 15 nm) is composed ofmyosin 31 (Fig. 5.4). Myosin filaments produce the A bands.
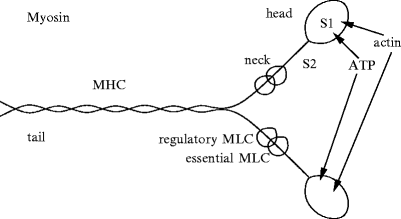
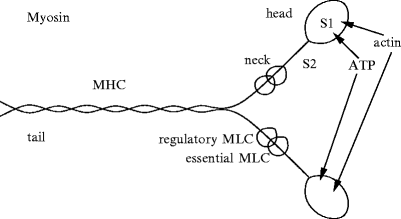
Fig. 5.4
Myosin filaments (MHC: myosin heavy chain, MLC: myosin light chain). The neck has binding sites for light chains, and the head for ATP and actin. For example, myosin-2 consists of 2 head segments: S1, composed of heavy chains, connected to a long dimeric rod by a flexible coiled-coil segment S2 (S2δ in β-myosin-2). The coiled-coil light meromyosin rod tails of myosin-2 are packed side by side, whereas both heads point away from the myosin filament surface (or light meromyosin dimer axis). S1 subfragments act as ATP-driven motors, the conformation and actin affinity of which change according to the status of ATPase sites. Actomyosin cross-bridges use chemical energy liberated by the hydrolysis of cardiac actomyosin Ca2 + -stimulated MgATPase.
The myosin structure is commonly split into 2 regions, light (LMM) and heavy (HMM) meromyosin. The former represents part of the tail and is responsible for the assembling of myosin (Fig. 5.4). The latter contains the 2 heads and the remaining part of the tail. Heavy meromyosin is further divided into globular fragment-1 (S1) and rod fragment-2 (S2). The latter contains binding sites for essential and regulatory light chains (MLC1 and MLC2). The former (S1) hasATP hydrolysis and actin-binding domains; it has helical arrangements around the filament axis.
The thick filament also contains M-line proteins myomesin andmyosin-binding protein-C (cardiac isoform cMyBPc or MyBPc3), or C-protein, that crosslink myosins and the M-band part of titin. Myosin-binding protein-C maintains the width of myosin-containing thick filament, as it restricts them to 200 to 400 molecules each.
Titin is involved in the connections between the ends of myosin filaments and Z discs; it can span the entire sarcomere length. It controls both the length and elasticity of the sarcomere.
Myomesin acts for lateral registration. This structural protein of the M line assists titin and myosin in the maintenance of their three-dimensional structure.
Cardiac myosin isoform-5.1 has higher ATPase and contractile activities than isoform-5.3 [362]. Myosin isoforms may have a heterogeneous regional distribution.
5.3.3 Actin Thin Filaments
The thin filament (diameter ∼ 5n nm) is composed ofactin, with small amounts of 2 regulatory proteins, troponin (TN) and tropomyosin (TMy, ).32 Actin filaments extend in direction normal to the Z lines from each side. They create the I band where they do not overlap the thick filaments.
Heterodimerictroponin is composed of 3 subunits: TnC, TnI, and TnT. Troponin-C has a binding site for Ca2 + (calcium receptor/handling protein). Troponin-I33 inhibits actin–myosin binding in the absence of Ca2 + ion. Troponin-I is regulated by TnC that relieves the inhibition once TnC binds Ca2 + . Troponin-T is a tropomyosin-binding protein. The troponin-C–Ca2 + complex deforms TnC–TnI element and weakens TnI–TnT, exposing the myosin-binding site on actin.
Troponin-I and myosin-binding protein-C are substrates ofprotein kinase-A. As it phosphorylates troponin-I, PKA reduces the Ca2 + sensitivity, but not the cross-bridge cycling rate [364].Protein kinase-C targets troponin-I, troponin-T, myosin light chain-2, and myosin-binding protein-C. Protein kinase-C may reduce the cross-bridge cycling rate.
Two actin polymers placed side by side form a double helix that behaves like a moving rack (Fig. 5.5). The screw-shaped myosin has a twisted tail and a head that emerges to form grooves in which the actin filaments slide like a wrench jaw.
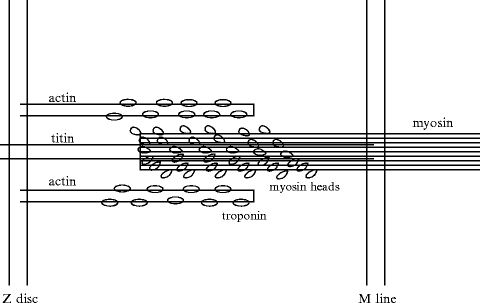
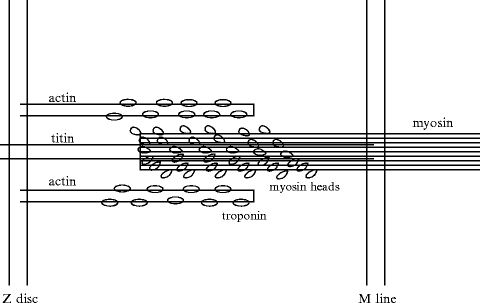
Fig. 5.5
Interacting myosin and actin in the sarcomere. The thick filaments are composed of myosin and its partners (myosin-binding proteins-C, -H, and -X). The thin filaments contain cardiac actin, α-tropomyosin, and TnT, TnI, and TnC troponins. Myocardium contraction is generated by cyclic interactions between actin and myosin. Conformational changes in the actin–myosin binding interface are related to ADP release and ATP binding. Nanoscale conformational changes are converted into macroscale myocardium displacements. The dissociation rate of actomyosin bonds was postulated to depend on strain in the Huxley model. The lifetime of the actomyosin bond indeed depends on the instantaneous load and loading history.
5.3.3.1 Actin–Myosin Interactions
Contraction is induced by the 4-time nanomotor composed of interdigitated myosin and actin filaments (Fig. 5.6) [365]: (1) the myosin head detaches from actin and fixesATP; (2) ATP is hydrolized and the myosin head binds toactin; (3) the myosin head releases the phosphate and undergoes a conformational change. Actin displacement is generated by a bascule motion of the myosin head that pulls on the actin filament. The myosin head rotates with an angle of about 45 degrees. This rotation leads to an actin displacement of about 10 nm. (4) The myosin releasesADP and remains anchored to actin. The interaction force is equal to about 1 pN [366]. Interdigitated actin and myosin filaments slide over each other to shorten the sarcomere during contraction (sliding-filament model).
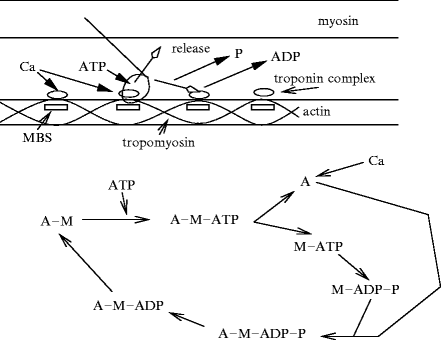
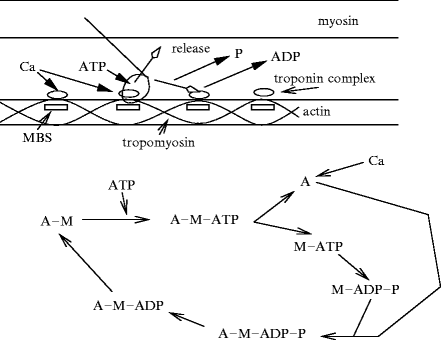
Fig. 5.6
Sliding-filament model of actin–myosin interactions: actin and myosin fixation, sliding, and detachment cycle (nanomotor cycle). In the absence of Ca2 + , tropomyosin locks the myosin-binding sites of actin. When Ca2 + binds to the troponin complex, troponin and tropomyosin free the mysosin binding site (MBS) of actin. The myosin head then binds to actin, forming a cross-bridge. Myosin detachment from actin is caused by ATP binding. ATP hydrolysis induces a rotation and a weak then strong rebinding of myosin to adjoining myosin-binding site of actin, associated with release of phosphate (P) and then ADP (phosphate release induces a rotation in myosin head). ADP unbinding leads to a new cycle if Ca2 + and ATP are available.
As a sarcomere contracts, Z lines are closer, and the widths of the I bands and H zone decrease, whereas the width of the A band remains constant. Conversely, as a myofibril is stretched, the width of the I bands and H zones increases (constant A-band width). Sarcomere shortening generates myofibril and muscle shortening.
Each molecule of myosin contains a myosin head that is a binding site for actin and ATP (Fig. 5.7). Troponin and tropomyosin allow actin to interact with myosin heads in the presence of Ca2 + ions. Activation of the muscle fiber causes the myosin heads to bind to actin. Actin is drawn a short distance ( ∼ 10 nm) past myosin. When linkages break, bonds are reformed farther along actin to repeat the process.34 The sarcomere length is maximum near the endocardium [367].
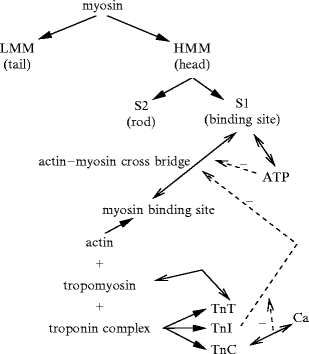
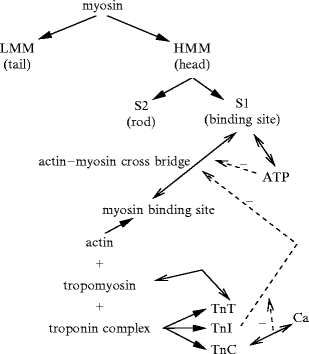
Fig. 5.7
Actin and myosin filaments. The thick filament is composed of many myosin units lined up in a bidirectional arrangement, each unit being made of 2 myosin chains wrapped around each other like a twisted golf club. The combination of calcium ions to troponin-C (TnC) that liberates the myosin-binding site on actin, followed by the inactivation of the tropomyosin–troponin complex, allows myosin head displacement and actin sliding. The heterotrimeric troponin complex is formed by regulatory troponin-C, inhibitory troponin-I (TnI), and tropomyosin-binding troponin-T (TnT). It is positioned along the actin filament at every seventh actin monomer. Troponin-C and calmodulin are the main targets of calcium ions. Both TnC and Cam contain 4 Ca2 + -binding domains. Calcium binding to the regulatory site of the cardiac troponin-C (cTnC) affects both its interaction with TnI and the interaction of TnI with TnT and tropomyosin. In the absence of Ca2 + , TnI inhibits the interaction of myosin-2 with actin. Ca2 + binding to cTnC relieves the TnI inhibition, ensuring contraction. Ca2 + -induced conformational changes of troponin-C are imparted to all actin monomers via TnI and TnT acting via 2 tropomyosin threads on both sides along the entire actin filament. cTnI phosphorylation by either PKA or PKC lowers the TnC affinity for calcium ions (positive lusitropy). Two main mechanisms change contractibility: (1) modifications in the amplitude and/or duration of transient Ca2 + fluxes and (2) modifications in the sensitivity of the contractile filaments to calcium by phosphorylation of the myosin light chain and/or troponin-I. Moreover, sarcomere compression due to stretching associated with rising diastolic filling brings the contractile filaments closer together, thus facilitating actin–myosin interaction and increasing the TnC affinity for calcium.
5.3.3.2 Myofibrils Scaffolding
5.3.3.3 Costamere
Myofibrils are held in position by scaffolds ofdesmin filaments, anchored by costameres, the cortical parts of the cytoskeleton, enriched invinculin 35 along the sarcolemma (Fig. 5.8). The costameres maintain the spatial structure of sarcomeres and couple the cardiomyocytes to the extracellular matrix. The membrane skeleton is made from spectrin and dystrophin, adapting the sarcolemma to CMC functioning and contributing to the force transmission. The costameres, membrane skeleton, and cytoskeleton are linked to the extracellular matrix by integral membrane proteins such as integrins, dystrophin–glycoprotein complexes and β-dystroglycan–laminin bounds. Dystrophin and β-dystroglycan are membrane proteins that are distributed in the sarcolemma andT tubules.
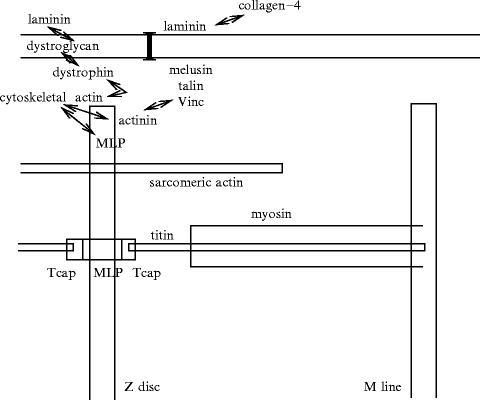
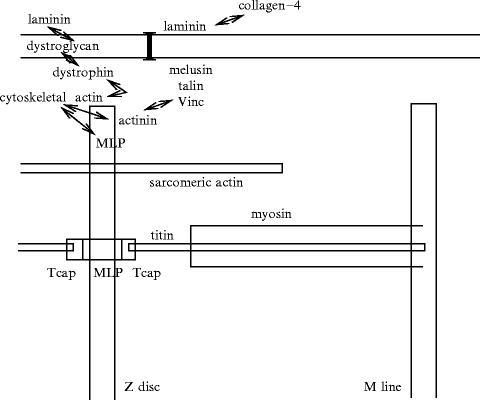
Fig. 5.8
Costamere is the junctional structure connecting the sarcomere to the sarcolemma and extracellular space. Costameres contain 3 main proteic complexes: (1) focal adhesions, (2) spectrin-based complex, and (3) dystrophin-associated complex. Costameric complexes, like the proteic complex of Z disc, contain α-actinin and muscle LIM protein (MLP). α-Actinin binds directly or indirectly vinculin (Vinc), talin, and melusin (a possible stretch sensor that links the costamere to integrins), among other proteins. α-Actinin is thus connected to the sarcoplasmic domain of muscle-specific β1D-integrin (I). Integrin extracellular domain links to laminin. The costamere hence coordinates the deformation of the sarcomere, sarcolemma, and extracellular medium (telethonin). In addition, dystrophin binds actin as well as the dystrophin-associated protein complex of the sarcolemma and sarcoplasma (dystrobrevin, dystroglycans, sarcoglycans, sarcospan, and syntrophins). Integral membrane proteins interact with components of the extracellular matrix via α/β-dystroglycan–α2-laminin complexes. Therefore, dystrophin also connects the sarcomere to the sarcolemma and extracellular matrix. Focal adhesions are composed of sarcoplasmic proteins (i.e., paxillin, talin, tensin, vinculin, and zyxin) that connect with cytoskeletal actin filaments and transmembrane proteins (α- and β-dystroglycans, α-, β-, γ-, and δ-sarcoglycans, dystrobrevin, and syntrophin).
5.3.3.4 Myosin-Binding Protein-C: Actin–Myosin Connector and Speed Reducer
The cardiac isoform ofmyosin-binding protein-C MyBPc3, a thick filament-associated sarcomeric protein member of the immunoglobulin superclass, is located in C zone of A bands.
Cardiac MyBPc binds to actin, myosin, and titin filaments, thereby participating in the organization of the sarcomere structure. Modulator MyBPc3 of sarcomere contractility stabilizes actin in its filamentous state, links actin and myosin filaments, and modulates actin–myosin interaction [368].
Protein MyBPc occupies a portion of the overlap zone — C zone. It reversibly binds via its C-terminus to the myosin filament. Its N-terminus reversibly links to the actin filament, thereby creating a secondary actomyosin connection. It contributes to regulating contraction velocity [369].
The MyBPc3 N-terminus slows actomyosin motion in parts of the thick filament within the C zone. The cardiac isoform contains a domain adjacent to its N-terminus endowed with several serine residues. Contraction slowing is tuned by phosphorylation of 4 serines (Ser273, Ser282, Ser302, and Ser307) [369]. Dephosphorylation of MyBPc3 can lead to its proteolytic cleavage.
Phosphorylations of accessory protein MyBPc3 at different sites byCa2 + –calmodulin-activated kinases and protein kinasesPKA andPKC maintain thick filament spacing [370]. β-Myosin-2 flexible coiled-coil segment S2δ has a charge distribution with 3 prominent rings of negative potential [371]. Charge interactions can regulate cardiomyocyte activity via myosin-binding protein-C bound to S2 [372].
Adrenergic stimulation of the myocardium leads to quick phosphorylation of the cardiac isoform of myosin-binding protein-C by protein kinase-A, as well as to phosphorylation of troponin-I andphospholamban. These phosphorylations contribute to the positive inotropic effect of adrenergic substances. Phosphorylation of MyBPc3 decreases during ischemia–reperfusion injury and pathologicalhypertrophy (Vol. 6 – Chap. 7. Heart Pathologies), reducing the binding quality between actin and myosin.
5.3.4 Z Disc, a Stretch-Sensing Structure
The structure of sarcomeres is highly ordered. Nonetheless, sarcomeres permanently exchange molecules with surrounding cellular structures. The cytoskeletal support system surrounds and supports myofibrils.
The Z discs not only serve as crosslinkers of thin filaments and transmitters of force generated by the myofilaments, but also as stretch receptors and signal transducers [373]. Various signaling molecules interact with Z-disc proteins. Several interacting substances shuttle between the Z disc and other cellular compartments.
The Z discs are mainly composed ofα-actinins assembled in successive layers (width ∼ 20 nm) that provide a skeleton for the insertion of actin filaments, titin, and nebulin (Fig. 5.9). Z discs of neighboring sarcomeres are aligned in parallel and connected via desmin. The sarcomere is coupled to the sarcolemma by costameres that comprise peripheral Z-disc molecules and subsarcolemmal proteins. Z disc and costameric proteins are involved in mechanotransduction.
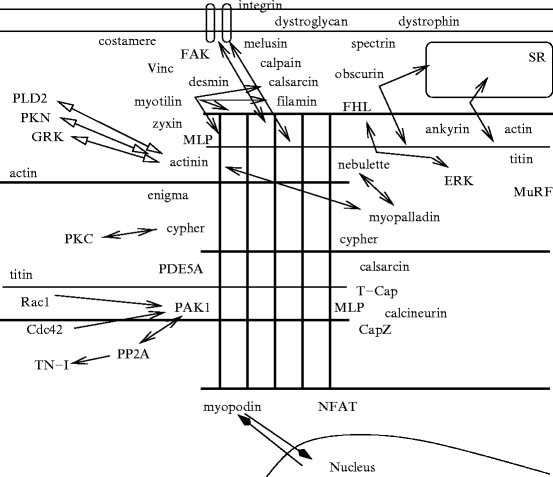
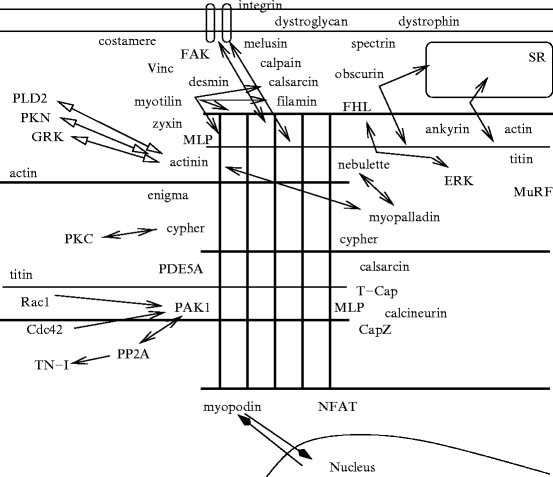
Fig. 5.9
Z-disc proteins and partners, and their interactions (Source: [373]). Z disc is a lattice of interdigitating proteins, such as nebulette, myopalladin, myotilin, filamin, CapZ, cypher, myozenin-1, etc. This proteic complex particularly contains α-actinin, titin-cap (T-cap or telethonin), muscle LIM protein (MLP, CLP, or CSRP3) that binds to actin and titin. Titin extends over half a sarcomere. Titin crosses the Z disc. Titin then associates the Z disc to the M line, both serving as sarcomere scaffolds (supporting and organizing actin and myosin filaments in parallel arrays by crosslinking antiparallel actin and titin). Desmin filaments surround the Z disc, connecting it to cortical (subsarcolemmal) costameres. The sarcomere is also linked to costameres via sarcomeric α-actinin and non-sarcomeric actin (i.e., γ-actin).
5.3.5 Main Sarcomeric Constituents
5.3.5.1 α-Actinin
α-Actinin-2 is the main component of the Z disc. α-Actinin-2 forms homodimers aligned in an antiparallel fashion. It contains 3 domains: actin-binding sequence, central rod, and domain with 2 calcium-binding motifs. α-Actinin-2 interacts with multiple molecules, such as the Rho effector protein Ser/Thr kinasePKN,phospholipase-D2,36 andG-protein-coupled receptor kinases.37 α-Actinin interacts with Z-line filamentous actin-capping protein (CapZ). Dimer CapZ is composed of α and β-subunits.
5.3.5.2 Titin
Titin, the largest human protein, spans the sarcomere from the M line to the Z line, where it is anchored. Titin serves not only as a structural component, but also as astrain sensor using its C-terminal protein Ser/Thr kinase domain at M line38 to adapt myocyte to changes in strain.
The titin kinase domain bears self-inhibition of its C-terminal regulatory tail that blocks theATP-binding site as well as auto-inhibition of the catalytic domain. Strain provokes a conformational change that removes the C-terminal auto-inhibitory tail, thereby allowing access to the ATP-binding site and simultaneously relieving auto-inhibition by partial unfolding that stimulates the kinase domain for subsequent autophosphorylation [376].
Titin is responsible for myocyte elasticity by acting as a spring for sarcomere retraction of stretched cardiomyocytes. Titin near-Z-disc and near-M-line segments bind to actin and myosin and myosin-binding protein-C MyBPc3, respectively. In the relaxed state, titin I-band segment is highly folded [377]. When the sarcomere is stretched, titin extensible I-band segment elongates and develops a tension. During contraction below slack length, myosin filaments move into the near-Z-disc segment of titin that then generates a restoring force useful for diastolic filling. Titin I-band segment is composed of many subsegments of different rheological properties. Among these subsegments, N2a and spring element N2b in the cardiomyocyte sarcomere determine titin isoforms (N2b and N2ba). Titin subtype N2ba contains both N2a and N2b segments. Cardiomyocytes coexpress long (N2ba) and short (N2b) titin isoforms in half-sarcomeres. The N2ba-to-N2b ratio changes to adjust stiffness.39 The A-band and near-Z-disc segments are inextensible.
Titin N2b subsegment interacts with members of the LIM family that bindcreatine kinase andadenylate cyclase for appropriate compartmentation. Titin N2b subsegment is a substrate for protein kinase-A [378]. Ventricular stiffness may then be modulated by adrenergic signaling via titin phosphorylation.
Calcium binding to titin, especially to N2ba, also affects the titin rheology. Calcium also alters interactions between titin and actin.Ca2 + -binding protein S100A1 that exists in high concentration in the myocardium inhibits the interaction between titin and actin.
5.3.5.3 Telethonin
Titin crosses the Z disc up to its binding partnertelethonin, or titin-cap (T-cap), at the periphery of the adjacent Z disc. Telethonin links titin to signaling and structural molecules, such as delayed rectifier K + channel in the T tubule and smallankyrin-1 that couples the T tubule to the sarcoplasmic reticulum. Titin also binds obscurin that associates with ankyrin.
Interactions between the Z-disc segment and various compounds may act in the positioning of the sarcoplasmic reticulum and T tubule close to the I band and ensure structure mobility according to sarcomere activity.
Telethonin also interacts withcysteine- and glycine-rich protein CSRP3,40 a nuclear regulator of myogenic differentiation and possibly a stretch sensor that mediatesnatriuretic peptide release.
5.3.5.4 Obscurin
Obscurin is a large ( ∼ 820 kDa) cytoskeletal calmodulin- and titin-interacting protein. It contains 68 immunoglobulin, 2 fibronectin-3, 1 calcium–calmodulin-binding (IQ motif), 1 SH3, 1 DH, 1 RhoGEF (Rho guanine nucleotide-exchange factor), 1 PH, and 2 Ser/Thr kinase MLCK-like domains [379, 380]; hence, its other names RhoGEF30 and obscurin
. Multiple immunoglobulin and fibronectin domains enable interactions with myosin and other sarcomeric and cytoskeletal components. Its RhoGEF domain interact selectively with RhoA at the M band and activate this small GTPase [381]. Its Ig domain-binding sites mediate self- and hetero-assembly. They particularly connect to titin as well as slow isoform-1 of myosin-binding protein-C (MyBPc1) and myomesin [380].

Obscurin pertains to the family of giant sarcomeric signaling proteins that includes titin and nebulin that are needed for the assembly of myofibrils and the structural integrity of the sarcomere. Some titin isoforms also contain a protein kinase domain similar to that of myosin light chain kinase. Members of the MLCK family act in the regulation of actin–myosin organization, especially sarcomere assembly.
This protein of striated myocyte concentrates at M bands and Z discs of the sarcomere, where it can sense contractile activity. However, obscurin localizes preferentially to the M band rather than the Z disc [380].
Several obscurin isoforms ( ∼ 70–870 kDa) are encoded by the single OBSCN gene. Alternative splicing generates: (1) conventional obscurinGEF 41 that contains only the GEF domain ( ∼ 800 kDa) and (2) kinase domain-containing obscurinMLCK ( ∼ 900 kDa).42 Smaller obscurin isoforms that possess the RhoGEF domain accumulate at the Z disc.
In addition, in normal epithelia, obscurins localize to cytoplasmic puncta, plasma membrane, and nucleus (110- and 120-kDa nuclear isoforms) [382].
Titin is connected to the sarcoplasmic reticulum by the giant muscular protein obscurin andankyrin. Obscurin also binds to calmodulin in a calcium-independent fashion. It possesses also binding sites for different isoforms of ankyrin-1 and -2 from the sarcoplasmic reticulum as well as signaling mediators, in addition to sarcomeric proteins. In addition, obscurin and ankyrin regulate ryanodine channel distribution.
Striated muscle preferentially expressed gene product (SPEG)43 is closely related to obscurin, with which it shares Ser/Thr kinase MLCK-like domains.
Obscurin helps to keep extrasarcomeric structures in lateral alignment with the sarcomere during contraction–relaxation cycles. Moreover, obscurin-related proteins such as obscurin-like protein-1 (ObsL1) serves also as cytoskeletal adaptor. Protein ObsL1 links the cytoskeleton to the cell membrane, particularly at sites of between-cell and cell–matrix adhesions [383]. Multiple isoforms arise from alternative splicing. Agent ObsL1 localizes to intercalated discs, perinuclear region, and M and Z lines. This cytoskeletal linker positions and stabilizes intercellular contacts and organelles within the cytoskeletal framework.
5.3.5.5 Nebulette and Filamin and Its Partners
Nebulin is an actin-binding protein.Nebulette is the cardiac-specific small isoform of nebulin.Filamin-2 46 is the filamin isoform specific to striated myocyte. Filamin-2 binds to several partners, such as calsarcin, myotilin, sarcoglycan, and β1 integrin.
Myotilin binds to α-actinin-2, filamin-2, and actin. It also interacts with calsarcin-1 and -2.Myopalladin interacts with manifold proteins, such as nebulette, α-actinin, and cardiac ankyrin repeat protein.Myopodin 47 moves from Z discs to the nucleus.
5.3.5.6 Members of the PDZ–LIM Family
The PDZ–LIM protein family48 contains 10 known members that serve as scaffold proteins. These cytoplasmic proteins bind to the cytoskeleton viaα-actinin. The PDZ–LIM family can be subdivided into 3 classes according to the number of LIM domains (Table 5.4). PDZ and LIM domain-containing protein PDLIM1 possesses a single C-terminal LIM domain. On the other hand, PDLIM5 (or Enigma homolog) and PDLIM7 (Enigma) contain 3 LIM domains. In the heart, PDZ–LIM family members act as adaptors that connect mechanical sensors embedded in specialized cytoskeletal structures such as the Z disc, to signaling mediators.
Table 5.4
The PDZ–LIM family of scaffold proteins or kinases. In the heart, members of this family intervene during embryogenesis as well as adaptive remodeling in response to mechanical stresses and strains.
Type | Alias(es) |
---|---|
Class-1 | |
PDLIM1 | Elfin, CLIM1, CLP36 |
PDLIM2 | Mystique, SLIM |
PDLIM3 | ALP |
PDLIM4 | RIL |
LMO7 | FBx20 |
Class-2 | |
LIMK1 | |
LIMK2 | |
Class-3 | |
PDLIM5 | EnH (EnH1) |
PDLIM6 | Cypher, ZASP, LDB3, Oracle |
PDLIM7 | Enigma, LMP1 |
LMP4 |
5.3.5.7 Categories of PDZ–LIM Proteins
Group-1 PDZ–LIM proteins that have a single PDZ domain and a single LIM domain include: (1) PDLIM1,49 (2) PDLIM2,50 (3) PDLIM3,51 (4) PDLIM4,52 and (5) LIM domain-only protein LMO7.53 Actinin-associated LIM protein is expressed at lower levels in the heart than skeletal muscles.
Group-2 PDZ–LIM proteins are represented by 2 protein kinases:LIM kinase LIMK1 and LIMK2. They contain a PDZ domain, 2 LIM regions, and a Tyr kinase sequence.
5.3.5.8 Enigma Group
In humans, 4 spliced variants of the Enh transcript produce 4 isoforms (EnH1–EnH4). Isoform EnH1 is expressed in embryonic and neonatal hearts, whereas, in adults, EnH2 to EnH4 are predominant [384]. Isotype EnH1 possesses an N-terminal PDZ domain and 3 C-terminal LIM domains, whereas EnH2 to EnH4 do not have LIM domains, but retain the N-terminal PDZ domain.
Enigma is able to bind actin, β-tropomyosin, and protein kinases. Agent EnH1 is an adaptor forprotein kinase-C. It also links toprotein kinase-D1 to regulate the activity of CaV1 channels. In normal adult hearts, EnH4 does not recruit PKC and PKD enzymes. During sustained arterial hypertension, EnH1 can be re-expressed and scaffold PKC and PKD kinases [384]. Subtype EnH1 promoteshypertrophy, whereas other isotypes prevent hypertrophy.
Cypher interacts also with α-actinin-2. Its isoform Cypher-2 binds to various, but not all types of protein kinase-C.
LIM–PDZ domain-containing protein LMP4 is coexpressed with and directly binds to heart-specific T-box TBx5 transcription factor. Unbound to LMP4, Tbx5 shuttles between the nucleus and cytoplasm; in a complex with LMP4, it localizes to actin filaments [385]. The TBx5–LMP4 complex undergoes assembly–disassembly cycles in response to external stimuli. Protein LMP4 represses TBx5 activity.
5.3.5.9 Four-and-a-Half LIM-Only Proteins
The family of four-and-a-half LIM (abnormal cell lineage LIN11, insulin gene enhancer protein Islet-1 (Isl1), and mechanosensory abnormality protein MEC3)-only proteins (FHL), also named skeletal muscle LIM domain-containing proteins (SLIM), includes FHL1, or SLIM1,57 FHL2, or SLIM3,58 and FHL3, or SLIM2.59 Four-and-a-half LIM domain-containing protein is a LIM-only protein that is characterized by 4 LIM domains (double zinc finger sequences) and a single zinc finger domain (LIM half-motif) in the N-terminus. Members of the FHL family can homo- or heterodimerize.
Members of the FHL family are regulators of cell differentiation and remodeling of the cytoskeleton. The FHL proteins act in extracellularsignal-regulated kinase signaling. In addition, at least some FHL proteins are transcriptional coactivators, but with distinct affinity for CREB and CREM transcription factors [386]. These members of the subclass-1A basic helix–loop–helix leucine zipper (bHLHzip) transcription factors regulate proliferation and differentiation of various cell types. They homodimerize and heterodimerize (CREB–CREM heterodimers) and then can connect to the cAMP-responsive element (CRE) in the regulatory region of diverse target genes.60
Protein FHL2 can associate with α
-, α
-, α
-, and several β-integrin subunits.



5.3.5.10 LIM-Only Protein Zyxin
Zyxin, a ubiquitous member of the LIM-only protein family, is involved in cytoskeletal organization. It is mainly located in focal adhesion complexes close to the Z disc. It binds to α-actinin and cardiac (muscle)-specific LIM protein (MLP or CLP),61 a member of the C-reactive protein family that belongs to the cardiomyocyte cytoskeleton.
5.3.5.11 Muscle/Cardiac-Specific LIM Protein
The Z disc of cardiomyocytes contains a stress sensor made ofmuscle-specific LIM protein, titin-cap, and titin. Cardiac LIM protein binds to α-actinin, telethonin, nebulin-related actinin-binding protein NRAP,PP3 phosphatase, and β-spectrin. Cultured cardiomyocytes deficient for MLP do not express natriuretic peptides ANP and BNP in response to stretch.
5.3.5.12 Calsarcins
Calsarcins are calcium–calmodulin-dependent phosphatases, PP3-associated sarcomeric proteins that are involved in cardiac hypertrophy. Calsarcin-1 is exclusively expressed by the adult myocardium and slow-twitch skeletal muscle, calsarcin-2,62 and calsarcin-3 by fast-twitch skeletal muscle fibers. Calsarcins interact with α-actinin, telethonin, filamin-2,cypher, and myotilin.
5.3.5.13 PP3 Phosphatase
Phosphatase PP3, or calcineurin, a calcium–calmodulin heterodimer, interacts with calsarcin-1 at the Z disc. It induces myocardial hypertrophy (Sect. 5.8). It dephosphorylates transcription factors of the NFAT family for translocation into the nucleus. Cytoplasmic PP3-dependent NFATs can be detected at the Z disc. After dephosphorylation by PP3, nuclear translocation of NFAT3 allows coactivation of muscle-specific adenylosuccinate synthetase AdSS1 gene [387].63 Electrical pacing of cultured cardiomyocytes that serves as a model of cardiac hypertrophy activates muscle-specific Adss1 gene, but represses non-muscle Adss2 gene.
5.3.5.14 ARGBP2
Ubiquitous Abelson-related gene product (ARG)-binding protein-2 forms a complex withCBL ubiquitin ligase, a regulator of the actin cytoskeleton and apoptosis.64 Agent ArgBP2 associates with and is a substrate of cytosolic Tyr kinases of the Abelson family Abl1 and Abl2 (or ARG).
Protein ArgBP2 abounds in cardiomyocytes, where it localizes to Z discs of sarcomeres. This adaptor assembles signaling complexes at Z discs. In particular, ARG-binding protein-2 binds to α-actinin at Z discs. It influences the contractile or elastic properties of cardiac sarcomeres.
5.3.5.15 Calpains
Calcium-dependent cysteine peptidases,calpains, target sarcomeric proteins and focal adhesions during cell migration. Calpain-3, the isoform specific to striated muscle, is located at the Z discs, costameres with desmin and ezrin, the M line, and the I band. Calpain-3 activates NFκB.
5.3.5.16 Desmin
Desmin, a muscle-specific intermediate filament protein, links the costameres to the Z disc. It is a component of the intercalated disc in cardiomyocytes. Desmin interacts with nebulin, spectrin,ankyrins, calpain-3, and the intermediate filament proteins synemin and syncoilin.
5.3.5.17 Integrins
Integrins couple the cytoskeleton, especiallycostameres, to the extracellular matrix. Integrins also link to other cell adhesion-associated signaling proteins and operate as mechanotransducers. Prolonged mechanical loading of the myocardium causes maladaptivehypertrophy as well as changes in expression of integrin and extracellular matrix proteins. Mechanical stress applied to integrins elevates cytoskeletal stiffness.
Sarcolemmal α1– and α2-integrins, expressed in the embryonic heart, can be re-expressed in an adult heart bearing unusual mechanical loading. Integrin-β1D is specific to cardiomyocytes and skeletal myocytes. Integrins associate withfocal adhesion kinase,integrin-linked kinase and its partner affixin, calreticulin, and melusin.
Cardiomyocytes synthesize at least 4 integrin types: α1β1-, α3β1-, α5β1-, and α7β1-integrins. Integrins-α3β1 and -α5β1 interact with extracellular fibronectin. This interaction is modulated by the contractile state of cardiomyocytes [388].
5.3.5.18 Melusin
Muscle-specificmelusin, or integrin-β1-binding protein Itgβ1BP2 (encoded by the ITGB1BP2 gene), is expressed in skeletal and cardiac, but not smooth myocytes. It localizes to costameres in the vicinity of Z disc. It senses mechanical stresses. It promotes the phosphorylation (inactivation) ofglycogen synthase kinase GSK3β, thereby preventing transcription factor activity [389].
5.3.5.19 Some Types of Sarcomeric Enzymes
5.3.5.20 Protein Kinase-C
Protein kinase-Cε, located at the Z disc, regulates cardiomyocyte contractility andhypertrophy. Activated by GPCRs, PKCε binds anchoring proteins, receptors for activated C-kinases (RACKs). PKCε induces normal growth (with preservation of the cardiomyocyte contractility and without fibrosis), rather than maladaptive hypertrophy.
5.3.5.21 P21-Activated Kinase-1
P21-activated kinase-1, a Ser/Thr protein kinase activated by Rac1 and CDC42 GTPases, is located at the Z disc, sarcolemma, intercalated discs, and nuclear membrane. Kinase PAK1 interacts withprotein phosphatase-2, decreasing the phosphorylation of cardiac troponin-I, and enhancing calcium sensitivity.
5.3.5.22 Phosphodiesterase-5A
cGMP-specific phosphodiesterase-5A localizes to the Z disc. It dissociates from the Z disc during heart failure.
5.4 Cardiac Adenylate Cyclases and the cAMP Pathway
In cardiomyocytes as well as vascular endothelial and smooth muscle cells,cAMP second messenger is involved in signaling transduction by acetylcholine, adenosine, catecholamines, endothelin, glucagon, glucagon-like peptide-1, prostaglandins (PGe1 and PGe2), and urocortin, among others. Signaling compartmentalization and use of distinct effectors can explain distinct effects of cAMP signaling caused by different messenger types.
First messengers target G-protein-coupled receptors, which subsequently activatetransmembrane adenylate cyclase. Afterward, cAMP operates via 4 types of effectors: (1) protein kinase-A; (2) RapGEF3 and RapGEF4 (
GEFs or EPACs); (3) cyclic nucleotide-gated ion channels (CNG); and (4) phosphodiesterases (PDE). The latter catabolize cAMP into AMP; however, PDE10a can be inhibited by cAMP that acts either as a competitive antagonist or as an allosteric regulator [390].

Protein kinase-A contains 2 regulatory and 2 catalytic subunits. Once catalytic subunits are liberated, PKA phosphorylate multiple effectors, such as enzymes (e.g., glycogen phosphorylase), transcription factors (e.g., CREB), nanomotors (troponin-I, myosin-binding protein-C), ion channels (Ca
1.2, ryanodine receptor), and other signaling components.

On the other hand,soluble adenylate cyclase resides inside the nucleus and mitochondria, as well as at microtubules and centrioles. In mitochondria, it couples the electron transport chain with cellular respiration. It is activated by HCO3 − and/or calcium. Once they are internalized, plasmalemmal receptors of extracellular messengers can signal via soluble adenylate cyclase. The latter is involved in apoptosis in coronary endothelial cells and cardiomyocytes [390].
5.5 Calcium Signals
Cardiomyocyte growth and contractility are spatially and temporally regulated by a set of ion channels, pumps, and exchangers responsible forCa2 + shuttling between the cytosol, intracellular stores, and extracellular milieu.
5.5.1 Calcium-Dependent Ion Carriers
Calcium ion inactivates voltage-dependent calcium channel, but activates other ion carrier types. Calcium-activated ion channels in the heart include [391]: (1) calcium-dependent chloride channels, which can contribute to the initial repolarization; (2) calcium-activated non-selective monovalent cation channels, which carry inward current at negative potentials; and (3) small-conductance calcium-activated potassium channels (Table 5.5). Whereas the rapid delayed rectifier K + current (i K,r), which is involved in repolarization phase 3, may be purely voltage dependent, the slow delayed rectifier K + current i K,s is more efficient at high cytosolic Ca2 + concentration [391].
Table 5.5
Cardiac calcium-dependent ion fluxes upon increased cytosolic Ca2 + concentration (Source: [391]; APD: action potential duration;
: Ca2 + increases conductance of ion carrier;
: inhibition). Stronger Ca2 + transients generate longer and briefer action potentials when ionic currents are inward and outward, respectively. Because cardiomyocytes contain several types of calcium-activated or -modulated ion carriers, the net effect depend on the balance between these carriers.


Inward flux | Outward current and |
---|---|
reduced inward flux | |
(prolonged APD) | (shortened APD) |
Na + –Ca2 + exchanger ( ![]() | Ca ![]() ![]() |
Non-selective Ca2 + -gated | Slow delayed rectifier K + channel ( ![]() |
monovalent cation channel ( ![]() | Ca2 + -activated Cl − channel ( ![]() |
Small-conductance Ca2 + -activated | |
K + channel ( ![]() |
The cardiac action potential triggers opening ofCaV1.2 channels. Resulting Ca2 + entry subsequently causes Ca2 + release from the endoplasmic reticulum throughryanodine receptors. Calcium ions are removed from the cytosol, particularly by endoplasmic reticulum uptake viasarco(endo)plasmic reticulum Ca2 + ATPases (SERCA) and extrusion through the plasma membrane viaNa + –Ca2 + exchangers.
5.5.2 Inositol Polyphosphate Messengers – Phosphoinositide Sources
Therefore, whereas inositol (1,4,5)-trisphosphate is a major regulator of Ca2 + -based responses in non-excitable cells, excitable cells such as cardiomyocytes employ a coupled system of Ca2 + import (entry from the extracellular space and influx from the intracellular stores through ryanodine receptors) rather than Ca2 + release from the endoplasmic reticulum throughIP3R receptors.65 In ventriculomyocytes, IP3R2 localizes mainly in the perinuclear region and nuclear envelope [392]. In atriomyocytes, IP3R2 content is 6 to 10 times larger than in ventriculomyocytes. These receptors are detected in regions of the sarcoplasmic reticulum adjacent to the sarcolemma, near ryanodine receptors.
Nonetheless, in human cardiomyocytes, a slight (1.5- to 2-fold) overall elevation of PLC activity results from activation of α1-adrenergic or endothelin ETA receptors (20- to 50-fold increase in non-excitable cells in response to angiotensin-2 and acetylcholine) [392].
Inositol phospholipids, phosphatidylinositol, and its phosphorylated derivatives lodge in cardiomyocyte sarcolemma in concentrations similar to those in other cell types. Stimulated α1-adrenoceptors and ETA receptors in cardiomyocytes involve primarily the generation of I(1,4)P2n cleaved by PLC from PI(4)P agent [392]. Under normoxia, IP3 production is limited. Whereas the IP3 pool does not change significantly upon stimulation,66 concentrations of I(1,4)P2 and IP1 isomers67 increase markedly upon receptor activation [392]. Messenger IP3 is formed from PI(4,5)P2 byphospholipase-C. On the other hand, I(1,4,5)P3 is rapidly processed by I(1,4,5)P3n 3-kinases and membrane-linked I(1,4,5)P3 5-phosphatases that deactivate the IP3 signal.
Phospholipase-C has generally a low activity in the heart. Four widespread PLC isozymes exist (PLCβ–PLCε). Isoform PLCβ is activated by subunits of the Gq subclass or by Gβγ subunits of the Gi subclass members; PLCγ by receptor protein Tyr kinases; PLCδ by Ca2 + ; PLCε by the G12 subclass members.
5.5.3 Mitochondrial Calcium Uniporter
Mitochondrial Ca2 + n uniporter contributes to Ca2 + signal shaping. In adult cardiomyocytes, a fraction of mitochondria is apposed to terminal cisternae of the sarcoplasmic reticulum, where ryanodine receptors are located to generate Ca2 + -induced Ca2 + n release. Hence, these mitochondria can transiently experience high Ca2 + concentration during systolic, high-speed Ca2 + transients that can overcome the relatively low affinity of mitochondrial Ca2 + n uniporter for Ca2 + ion. In neonatal cardiomyocytes, the intramitochondrial Ca2 + level undergoes beat-to-beat oscillations; the average peak magnitude in mitochondrial Ca2 + n level is quite small ( ∼ 50% smaller than that in the cytoplasm [393]. At least in neonatal cardiomyocytes, mitochondria significantly contribute to buffering the amplitude of systolic cytosolic Ca2 + import [393]. Calcium peaks (20–30 μmol) are formed on the outer mitochondrial membrane during systole; overexpression of mitochondrial Ca2 + n uniporter significantly reduces them.
5.5.4 Mechanotransduction
Mechanical stretch during diastolic filling triggers mechanotransduction that regulates local production ofreactive oxygen species. A small diastolic stretch (8%) of a ventriculomyocyte indeed rapidly causes a burst ofCa2 + sparks due to the release of free Ca2 + n ions from intracellular stores. The microtubule network links stretching to changes in concentration of reactive oxygen species and intracellular free calcium ions. Cardiomyocyte stretching increases ROS production by sarcolemmal NADPH oxidase NOx2 using the microtubule network that then sensitizes nearby ryanodine receptors, thereby causing Ca2 + sparks [394].68 NADPH oxidase NOx2 localizes to the junctional sarcoplasmic reticulum, thereby allowing a rapid RyR2 oxidation and tuning RyR2 sensitivity to Ca2 + ion. Stretch-dependent oxidation of RyR2 actually is a rapid and reversible control mechanism of its Ca2 + sensitivity. However, in diseases, identical stretch creates transient cytosolic accumulation of free Ca2 + ions that can propagate through the cell and arrhythmogenicCa2 + n waves may result.
5.5.5 Sodium–Calcium Exchangers and Sodium–Potassium Pumps
In cooperation with plasma membrane Ca2 + ATPase (PMCA), sarco(endo)plasmic reticulum Ca2 + ATPase (SERCA), and Na + –K + –Ca2 + exchangers (SLC24a1–SLC24a6),Na + –Ca2 + exchangers (NCX1–NCX3, or SLC8a1–SLC8a3) enable recovery of intracellular calcium concentration to basal levels after stimulation. Subtype NCX1 that exchanges 3 extracellular Na + for 1 intracellular Ca2 + is ubiquitous. Both NCX2 and NCX3 are restricted to the brain and skeletal muscle. However, NCX2 is also expressed in aortic and intestinal smooth muscles. On the other hand, during cardiomyocyte depolarisation, Na + n–Ca2 + exchanger can operate in the reverse direction to allow calcium import and sodium export from the cytosol.
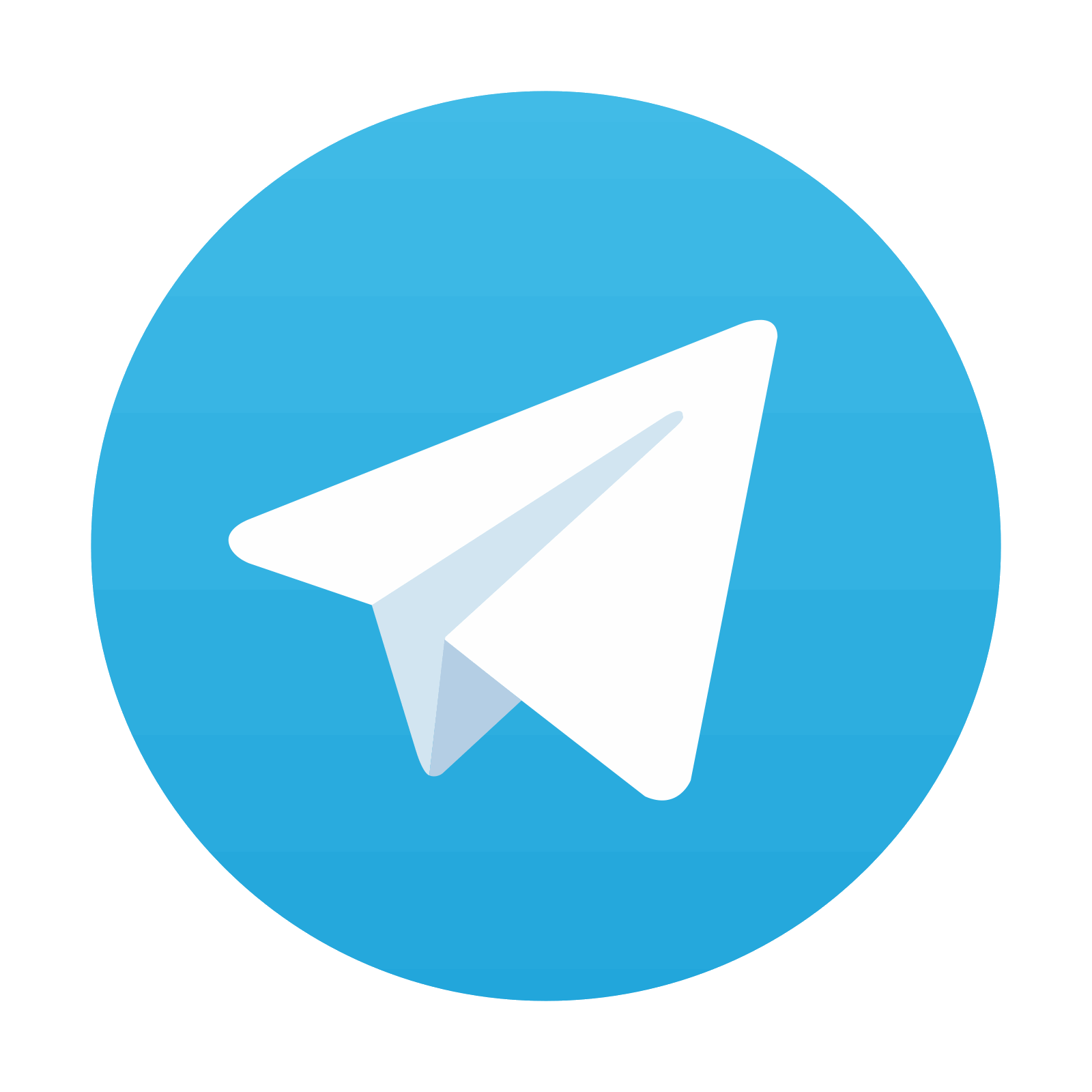
Stay updated, free articles. Join our Telegram channel
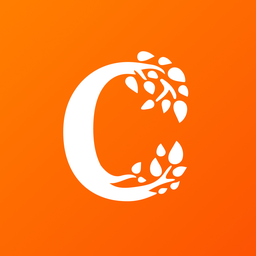
Full access? Get Clinical Tree
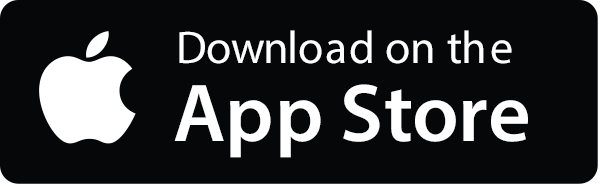
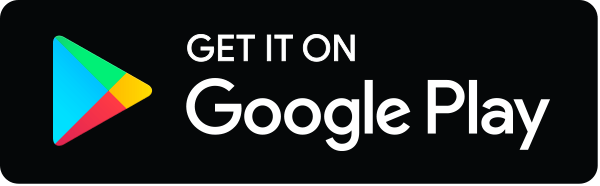