Fig. 27.1
Overview of the human immune system. Schematic highlighting the innate and adaptive immune system . Both arms play a major role in allograft survival and rejection. The innate immunity is the first-line nonspecific defense against nonself antigens. Its activation results in hyperacute rejection and can be generally avoided with ABO histocompatibility matching. The adaptive immunity is the dedicated and specific defense against nonself antigens. Activation of the adaptive immunity arm results in cellular and antibody-mediated rejection and is generally prevented or treated with chronic immunosuppression
The innate immune system includes more immediate and less specific responses not dependent on antigen recognition. It relies on the process of inflammation, humoral amplification, and phagocytosis to accomplish a rapid destructive reaction without a latent period required for lymphocytic activation and antibody production. When the circulation is restored in the transplanted heart, the innate immune system generates an inflammatory reaction induced by reperfusion of the transplanted heart. If left untreated, it will lead to allograft hyperacute rejection. The adaptive immune system , on the other hand, depends on responses mediated by T and B lymphocytes, requiring an interval following the first exposure of “nonself” before its destructive effects are manifested (◘ Fig. 27.2). Lymphocytes express antigen receptors that can recognize “nonself” antigens, culminating in cellular activation and antibody production.


Fig. 27.2
Humoral and cell-mediated immune responses to an antigen. An antigen is phagocytosed by a macrophage. The macrophage recognizes the antigen as “nonself” and forms a self–nonself complex that is mobilized to the cell surface of the antigen-presenting cell (APC). The APC presents the complex to the T-helper lymphocyte via a receptor-mediated process. Chemokines stimulate cellular proliferation of the T-helper lymphocytes and activation of B lymphocytes and T lymphocytes
Within minutes following reperfusion, the blood carries the donor materials to the spleen and lymph nodes. The antigen-presenting cells (APCs) within the spleen are capable of trapping large amounts of antigens and processes them for presentation to the T lymphocytes (◘ Fig. 27.3). The T lymphocytes migrate from the bone marrow (◘ Fig. 27.4) and peripheral circulation to the lymph nodes and spleen, enter the para-cortical areas, bind the major histocompatibility complex (MHC)-presenting antigens, and ignite the adaptive immune response and cellular-mediated rejection (CMR). In the process, T lymphocytes will interact with B lymphocytes. This interaction induces B-lymphocyte activation and differentiation into plasma cells that function in antibody production (◘ Fig. 27.5). This B-lymphocyte–T-lymphocyte interaction is the basis for antibody-mediated rejection (AMR) (◘ Figs. 27.1, 27.2, and 27.5).




Fig. 27.3
Histology of the human spleen . Histological sections of the spleen at low magnification (a) and at higher magnification (b). The spleen is a major lymphoid organ that is divided into the red pulp (rp), representing the storage of blood and the white pulp (wp), which are the lymphocytes, macrophages, plasma cells, etc. Note that the white pulp has a nodule (primarily containing B lymphocytes) that consists of a central germinal center and a peripheral mantle zone and an adjacent periarterial lymphatic sheath, which is the primary site for T lymphocytes

Fig. 27.4
Differentiation of the T lymphocytes . Schematic highlighting the maturation, activation, and the clonal expansion of the T lymphocytes. Hematopoietic stem cells and progenitors emerge from the bone marrow and populate the thymus. Subsequent maturity of the thymocytes ultimately produces cytotoxic, memory, suppressor, and T-helper lymphocytes

Fig. 27.5
B-lymphocyte-mediated production of antibodies. Nonself antigens are recognized by B lymphocytes and subsequently activate T-helper lymphocytes (via class II MHC-binding mechanism) or generate plasma cells that produce and release specific antibodies
Innate Immunity
Following the initial encounter with nonself, the nonspecific innate immunity is triggered (◘ Fig. 27.2). It primarily consists of macrophages, natural killer (NK) cells, granulocytes, and the complement cascade [6]. Morphologically, NK cells strongly resemble B or T lymphocytes; however, they are not antigen-specific and do not express T-cell receptors or immunoglobulins. NK cells function by lysing target cells or antigens regardless of the presence or absence of any major MHC complexes. Thus, they are directly involved with the initial nonspecific inflammatory response against the donor allograft.
Cytokines , on the other hand, are secreted proteins that alter the immunologic response of nearby cells in an autocrine and paracrine fashion during both innate and humoral responses. The major cytokines in heart transplantation include interleukin-2 (IL-2), interleukin-6 (IL-6), interferon-γ, and tumor necrosis factor (TNF). IL-2 is a key growth factor that is required for the expansion of T lymphocytes during a T-lymphocyte-mediated response (◘ Fig. 27.2). Stimulated cells begin to produce IL-2 and respond to IL-2 via an IL-2 receptor in an autocrine manner. IL-6 primarily induces B lymphocytes to differentiate into plasma cells. TNF is pro-inflammatory and cytotoxic and is primarily secreted by granulocytes. Interferon-γ mainly affects the differentiation of T lymphocytes into T-helper CD4 T cells. It also stimulates NK cells and CD8 T lymphocytes.
A critical step in the immune response pathway is the aggregation and transport of leukocytes into areas targeted for immunologic attack. The adhesion molecules play a key role in maintaining the structural integrity and promoting adhesion of leukocytes to surrounding structures. They include integrins, selectins, and immunoglobulin superfamily adhesion molecules. Adhesion molecules mediate the initial interaction of T lymphocytes with antigens, their migration, and retention within the transplanted organ. Likewise, the activation and migration of T lymphocytes in the lymph nodes and spleen are also dependent on adhesion molecules.
Generally, integrins act as cellular adhesive agents as they anchor cells to the extracellular matrix. Cell-to-cell adhesion is the role of selectins and immunoglobulin superfamily molecules. Although initial graft reperfusion following implantation causes host leukocytes to enter the graft, it also allows leukocytes and other cells in the donor organ to be “flushed” into the host. These cells travel through the blood to the peripheral lymphoid organs, including the thymus (◘ Fig. 27.6), nodes, and spleen (◘ Fig. 27.3), which are primary sites of the initiation of specific T-lymphocyte-mediated response (◘ Fig. 27.4).


Fig. 27.6
Histology of the neonatal human thymus . The histological structure of the neonatal thymus is shown at low magnification (a) and higher magnification (b). The thymus is dived into lobes and lobules (*) by septa. Each lobule is divided into the cortex (c), which is rich in T lymphocytes, and a central medulla (m), which contains epithelial cells and thymic corpuscles (tc). The thymus is fully developed prenatally and begins to atrophy following puberty
Another important arm of innate immunity is the complement system [7, 8]. As outlined in ◘ Fig. 27.7, the complement cascade can be activated through three different pathways: classical, lectin, and alternative. All three pathways of complement activation converge in the activation of complement component 3 (C3).


Fig. 27.7
Overview of the human complement system . Schematic highlighting the lectin, classical, and alternative complement activation pathways. The membrane-attack complex (MAC) is responsible for cellular lysis in the nonspecific innate immune response and hyperacute rejection
Activation of complement via the classical pathway is initiated by the first component of human complement (C1), which can bind to antibodies. When the classical pathway of complement is activated through an antibody-mediated process, the globular heads of a single C1 interact simultaneously with two or more Fc regions of IgM or IgG (◘ Fig. 27.8). Once C1 binds, its protease function is activated, and it cleaves the fourth component of complement (C4), yielding two fragments—C4a and C4b. C4 can also be activated by a mannose-binding lectin (MBL). After binding, the lectin can cleave C4 through two associated serine proteases (MASP-1, 2, and 3). C4b continues the complement cascade by binding to C2, which is then cleaved by C1 or MASP to generate C2b that diffuses away and by the remaining C2a that is associated with C4b. C4b and C2a together form the classical pathway C3 convertase (◘ Fig. 27.7). This complex is a protease that can cleave the central component of the complement system, C3.


Fig. 27.8
Schematic of the human antibody . Antibodies include the Fab arms that recognize antigens (light chain) and the Fc (heavy chain) components
C3 also can be directly activated by the alternative pathway of complement. Like C4, C3 contains an internal thioester group. In the alternative pathway, small numbers of C3 molecules sporadically undergo a spontaneous conformational change, and several of these C3 molecules covalently bind to nearby proteins. The microenvironment in which C3 binds determines whether this pathway continues through activation of factor B or whether factor H inactivates C3b. When factor B is bound to C3, it is a substrate for the serum protease factor D. Cleavage of factor B results in a soluble fragment Ba and a larger fragment Bb that remains complexed with C3. Properdin stabilizes the activity of the C3Bb complex, and this complex is the alternative pathway C3 convertase.
The classical and alternative pathways converge at C3. Either convertase can cleave C3 into two fragments, C3a and C3b. The smaller C3a fragment can bind to C3a receptors (C3aR) on nearby tissue mast cells, basophils, or eosinophils. The larger fragment, C3b, is deposited together with components of either the classical or alternative C3 convertase pathway forming a complex, the C5 convertase, which is capable of cleaving C5. The small fragment, C5a, is quantitatively much more potent as a chemotactic agent than C3a and affects a wider range of cells, including neutrophils, monocytes, basophils, and eosinophils. The larger fragment, C5b, initiates assembly of the terminal components of complement (C5b-C9) into a pore-forming structure, the membrane-attack complex (MAC). The MAC forms a hole in the membrane of the foreign allograft cells that disrupts membrane integrity and causes cell lysis. Hyperacute rejection is one of the manifestations of the speed and potency of complement activation by preformed antibodies directed against the transplanted allograft [9].
Adaptive Immunity
The specific adaptive response is primarily mediated by T lymphocytes, B lymphocytes, and APCs [10]. The cell surface proteins primarily responsible for the immune response in heart transplantation are called MHC or human leukocyte antigens (HLA ). T lymphocytes only recognize antigenic peptides that are contained within the MHC-binding domain on the surface of APCs. The MHCs are highly polymorphic, which establishes the basis for graft rejection. The function of MHC molecules is closely related to their three-dimensional structure, which is composed of two ɑ-helices on top of a β-pleated sheet.
The proteins of the MHC complex are subdivided into class I, class II, and class III. Class I and class II directly relate to transplantation but differ in the composition of the polypeptide chains that constitute them and in their distribution on different cells and tissues.
Class I molecules are expressed on all nucleated cells, with subtypes A, B, and C. The HLA–A gene includes more than 50 alleles, the HLA–B gene includes more than 75 alleles, and the HLA–C gene includes more than 30 alleles. These genes are composed of two chains—a larger, highly polymorphic, heavy ɑ-chain encoded on chromosome 6 and a smaller, non-polymorphic β-chain termed β-2 microglobulin encoded on chromosome 15. Antigens originating from inside the cell are processed via the endogenous pathways; they are complexed with MHC class I molecules and presented to T lymphocytes (◘ Fig. 27.2).
Class II molecules are expressed on a limited subset of cells, such as macrophages, dendritic cells, and B lymphocytes. The MHC complex classes that are relevant to transplantation are HLA-DR, HLA-DP, and HLA-DQ. These molecules are composed of an ɑ-chain and a β-chain. Unlike class I molecules, which interact primarily with CD8 T lymphocytes, class II molecules interact primarily with CD4 T lymphocytes. Antigens originating from outside the cells are processed via the exogenous pathway and presented with the MHC class II molecules, which is the principal mechanism for processing and presenting the alloantigens following heart transplantation [11]. ◘ Figure 27.9 demonstrates the three-dimensional structure of the class I and class II molecules.


Fig. 27.9
Structure of MHC I and MHC II proteins
T lymphocytes represent the cornerstone of the immune system that mediates the rejection response to the alloantigens that are expressed in the transplanted heart. The CD4 helper T lymphocytes function primarily to detect foreign antigens. Once activated, the CD4 helper T lymphocytes facilitate other cell types, such as CD8 cytolytic T lymphocytes, B lymphocytes, and neutrophils, to participate in the immune response (◘ Fig. 27.1). The CD8 cytolytic T lymphocytes function primarily as effector cells, which kill targeted cells expressing foreign antigens. During their development, the precursor T lymphocytes originate from the bone marrow (◘ Fig. 27.10) and then migrate to the thymus (◘ Fig. 27.6) where they first appear in the subcapsular cortex. Subsequently, they begin to express CD3, CD4, and CD8 molecules resulting in distinct T lymphocytes, and they acquire specific T-cell receptors needed to detect presented foreign antigens. During the selection process, the cells migrate from the thymic cortex to the medulla and then to the periphery (◘ Fig. 27.6).


Fig. 27.10
Histology of the human bone marrow . Low magnification demonstrating the fatty component (f) and erythroid and myeloid components
In the context of the immunologic response , the T-cell antigen receptor on the surface of T lymphocytes must first bind to the antigen presented by the MHC molecule. This binding then transmits a signal to the interior of the cell along the designated transduction pathways. This recognition process is very specific, despite the presence of many diverse antigens. This process recognizes antigens only in the presence of self-MHC molecules.
In the case of CD4 T lymphocytes, which initiate the cellular response to transplant antigens, the antigen must first be associated with an MHC complex on the cell surface through a process called antigen presentation . This presentation is performed by APCs (◘ Fig. 27.4). The antigen first must be internalized within the APC, where it is broken down into small polypeptides. The peptides are then physically associated with MHC molecules and exported to the cell surface as a complex. Cells that serve as APCs are dendritic cells, macrophages, and B lymphocytes. Dendritic cells are the most efficient in presentation and can be found scattered throughout the lymphoid and nonlymphoid tissues. After the recognition phase, the CD4 T lymphocytes progress through a series of activations that culminate in the induction of the rejection response. Specifically in cellular rejection, CD8 cytolytic T lymphocytes are jointly activated and programmed for nonself-destruction through exocytosis-mediated cytolysis and ligand-mediated triggering of apoptosis. Figure 27.11 shows the various transduction pathways involved (calcineurin and mTOR) that provide the basis for the immunosuppression agents being used.


Fig. 27.11
Overview of the functional impact of immunosuppression agents on the T lymphocytes. Note the impact of corticosteroids on the APCs: calcineurin inhibitors decrease the production of IL-2, MMF decreases purine synthesis, and sirolimus inhibits the mTOR pathway (ATGAM antithymocyte globulin, OKT3 antihuman CD3 antibody, MHC major histocompatibility complex, TCR T-cell receptor, IL–2 interleukin-2, IL–2R IL-2 receptor, NFAT nuclear factor of activated T cells, AP–1 activator protein-1, TOR/mTOR mammalian target of rapamycin, AZA azathioprine, MMF mycophenolate mofetil)
Similar to the T lymphocytes, the B lymphocytes originate or are produced in the bone marrow (◘ Fig. 27.10). However, they act via immunoglobulins, and the end products of differentiation for the B lymphocytes are plasma cells and memory B cells. B lymphocytes depend on surface immunoglobulins for the binding of antigens. Plasma cells produce immunoglobulins (antibodies) (◘ Fig. 27.5), that consist of four polypeptide chains in two pairs (◘ Fig. 27.8). Each pair of chains is identical to the other, consisting of a heavy chain and a shorter light chain. ◘ Figure 27.8 depicts the basic configuration of an antibody molecule.
In order for the immune system to contend with the vastly differing antigens, the antigen-binding portion of the antibody must be extremely heterogeneous (◘ Fig. 27.8). The heavy-chain regions form the basis of the classification of immunoglobulins into isotypes: IgG, IgA, IgM, IgD, IgE, and IgG [12]. The initial production of IgM and, later, IgG are the main isotypes involved in transplant rejection. The term humoral (or antibody-mediated) rejection refers to the production of antibodies against the nonself allograft cells, primarily mediated by the activation of B lymphocytes. The main effector mechanisms described in the humoral responses are neutralization, opsonization, and complement activation.
The discovery and characterization of such immune responses and their biological targets, as well as emerging classes of immunosuppressive therapies, have contributed to enhanced preservation of the transplanted allografts . Alteration of these biological mechanisms using agents such as steroids, antimetabolites, calcineurin inhibitors, mTOR pathway inhibitors and others have revolutionized the field of transplantation.
The History of Immunosuppressive Agents
Successful human transplants have a relatively long history that preceded the use of immunosuppression agents for postoperative survival and graft preservation. After decades of failed solid organ transplantation, in 1951, Medawar from the British National Institute for Medical Research suggested that immunosuppressive agents could be used to mitigate the effects of rejection [13]. Corticosteroid s had been traditionally used, and the more effective azathioprine (AZA) was identified in 1959, but it was not until the discovery of cyclosporine A (CsA) in 1970 that transplant surgery found a sufficiently powerful immunosuppressive (◘ Fig. 27.11).
In 1968, surgical pioneer Denton Cooley, MD, performed more than 15 transplants. Fourteen of these recipients did not survive more than 6 months [14, 15]. By 1984, two-thirds of all heart transplant recipients survived for 5 years or more. In 1981, the first successful heart–lung transplant took place at the Stanford University Hospital. The lead surgeon, Bruce Reitz, MD, credited the patient’s recovery to CsA [16]. Since then, many other drugs have been developed, such as the various calcineurin inhibitors, mammalian target of rapamycin (mTOR) inhibitors, and mycophenolate mofetil (◘ Figs. 27.11 and 27.12).


Fig. 27.12
Calcineurin inhibition by cyclosporine and tacrolimus. Cyclosporine and tacrolimus inhibit the phosphatase activity of calcineurin. This inhibition prevents the activation of NFATs and their translocation to the nucleus to activate IL-2 and other cytokines in the T lymphocytes (MHC major histocompatibility complex, FKBP FK-binding protein, IL–2 interleukin-2, NFAT nuclear factor of activated T cells)
Prospectively, the emerging field of regenerative medicine promises to solve the problem of organ transplant rejection by regrowing organs in vitro using allogeneic stem cells. Until then, the reliance on current pharmaceutical agents and the development of new agents remain vital for the continuation of transplant medicine.
Immunosuppressive Agents
Calcineurin Inhibitors: Cyclosporin A (CsA)
CsA is an immunosuppression drug widely used in organ transplantation for rejection prophylaxis. It interferes with the activity or expansion of T lymphocytes (◘ Figs. 27.11 and 27.12). CsA was initially isolated from the fungus Tolypocladium inflatum in 1969 [17]. It is a cyclic nonribosomal peptide of 11 amino acids and contains a single d-amino acid that is rarely encountered in nature.
The success of CsA in preventing organ rejection was initially demonstrated in kidney transplant recipients by Calne at the University of Cambridge [18] and in liver transplants by Starzl at the University of Pittsburgh [19]. It was then approved by the Food and Drug Administration (FDA) to prevent rejection of kidney, heart, and liver transplants. Mechanistically, CsA binds to the cytosolic protein cyclophilin (immunophilin) of T lymphocytes. This complex of cyclosporin–cyclophilin inhibits calcineurin which, under normal circumstances, is responsible for activating the transcription of IL-2 via dephosphorylation of nuclear factor of activated T cells (NFAT) transcription factors (◘ Fig. 27.12).
In T lymphocytes, the activation of the T-cell receptor normally increases intracellular calcium, which acts via calmodulin to activate calcineurin. Calcineurin then dephosphorylates the transcription factor, nuclear factor of activated T lymphocytes (NFATc), which translocates to the nucleus and increases the activity of genes encoding IL-2 and related cytokines (◘ Fig. 27.12). It also inhibits lymphokine production and interleukin release and, therefore, leads to a reduced function of effector T lymphocytes [20, 21].
CsA was first used in human cardiac transplantation in 1980 and then became the standard immunosuppressant in heart transplant recipients. No randomized prospective trials comparing the efficacy of CsA with conventional immunosuppressive regimens have been reported, and the rationale for its use emerged from preclinical animal models and kidney transplantation trials. However, the results of retrospective comparisons showed a marked improvement in outcome when patients were treated with CsA. For example, the 1- and 2-year survival rates were approximately 80 % and 70 %, respectively, in a single-center Stanford experience. These results represented a significant improvement in survival compared with the 60 % and 55 % 1-year and 2-year survival, respectively, formerly noted with AZA. Results reported by the University of Pittsburgh and the United Kingdom Trial were comparable, with 79 % and 76 % overall survival noted, respectively [22].
More recently, CsA has been used less frequently due to its extensive side effect profile and the presence of more effective immunosuppressive agents, such as tacrolimus and sirolimus (◘ Figs. 27.11 and 27.12). Nephrotoxicity represents the most frequent and clinically important complication associated with CsA use and may ultimately define the limits of clinical utility of the drug for long-term immunosuppression. CsA is primarily metabolized by the liver and excreted via the biliary system (◘ Table 27.1). It has a half-life of 8.4 h (◘ Table 27.1) [22].
Table 27.1
Current immunosuppressive agents used for cardiac transplantation
Drug | Therapeutic level | Major side effects |
---|---|---|
Steroids/prednisone | None—follow clinical response | Hypertension |
Diabetes | ||
Obesity | ||
Hyperlipidemia | ||
Osteoporosis | ||
Avascular necrosis | ||
Poor wound healing | ||
Cushingoid features | ||
AZA | None—follow clinical response | Anemia |
Thrombocytopenia | ||
Neutropenia | ||
GI toxicity | ||
Hepatic toxicity | ||
MMF | Controversial, trough levels of 2.5–5.0 g/mL | GI distress |
Anemia | ||
Thrombocytopenia | ||
Neutropenia | ||
CsA | Trough levels 300–350 ng/mL early, decreasing to ~200 ng/mL 1 year posttransplant | Hypertension |
Hyperlipidemia | ||
Renal insufficiency | ||
Tremor | ||
Hirsutism | ||
Gingival hyperplasia | ||
GI distress | ||
Hypomagnesemia | ||
Hyperuricemia | ||
Anemia | ||
Tac | Trough levels 10–15 ng/mL early, decreasing to 5–10 ng/mL posttransplant | Hypertension |
Diabetes | ||
Hyperlipidemia | ||
Renal insufficiency | ||
Osteoporosis | ||
GI distress | ||
Hepatic toxicity | ||
Hypomagnesemia | ||
Hyperuricemia | ||
Thrombocytopenia | ||
Sirolimus | Trough levels 5–15 ng/mL | Hyperlipidemia |
Poor wound healing | ||
GI distress | ||
Tremor | ||
Hyperuricemia | ||
Anemia | ||
Thrombocytopenia | ||
Neutropenia |
Tacrolimus (Tac)
Tac (FK 506) is a lipophilic 23-member macrolide lactone discovered in 1984 from the fermentation of the bacterium Streptomyces tsukubaensis. It was first approved by the Food and Drug Administration in 1994 for use in liver transplantation, and, since then, its use has been extended to kidney, heart, small bowel, pancreas, lung, skin, cornea, bone marrow, and limb transplants. Tac is a calcineurin inhibitor (via binding to FK 506-binding protein) with a similar mechanism of action as CsA, thus inhibiting both T-lymphocyte signal transduction and IL-2 transcription (◘ Fig. 27.12) [23]. It has been prospectively compared with CsA in three randomized trials, with no difference in 1-year survival or in the incidence of significant rejection [24–26].
Although the short-term impact of Tac and CsA are comparable regarding patient and graft survival, Tac has a more favorable lipid profile, increased potency, and is associated with a significantly lower rate of acute rejection compared with CsA-based immunosuppression [27]. This characteristic is unique, for it is well known that CsA will not reverse established ongoing immune responses. Tac is normally prescribed (Table 27.1) as part of a triple posttransplant regimen including steroids and mycophenolate mofetil (MMF). It is extensively metabolized in the liver by the CYP450 enzyme complex and is mainly excreted in feces (92 %) and urine (2 %) (◘ Table 27.1). Tac has a half-life of 4–40 h depending on the hepatic function (◘ Table 27.1).
One of the first major clinical trials that examined the efficacy of Tac in heart transplant recipients was performed at the University of Pittsburgh in 1992. At that time, it was used as the primary immunotherapy in conjunction with low-dose steroids and AZA. Overall 1-year patient survival was 92 %, with a low incidence of cardiac rejection episodes (grade III) within 90 days of transplantation (0.95/patient). Achieving this level of immunosuppression was comparable to that of CsA-based triple-drug therapy. Renal dysfunction occurred during the perioperative period in most patients in this trial. However, the incidence of hypertension was 54 % compared with 70 % during the CsA era. Ten adults underwent successful rescue therapy with Tac after cardiac rejection refractory to conventional immunotherapy [28]. Tac is now considered the standard of care for initiation and maintenance of immunosuppression for the cardiac transplant recipient.
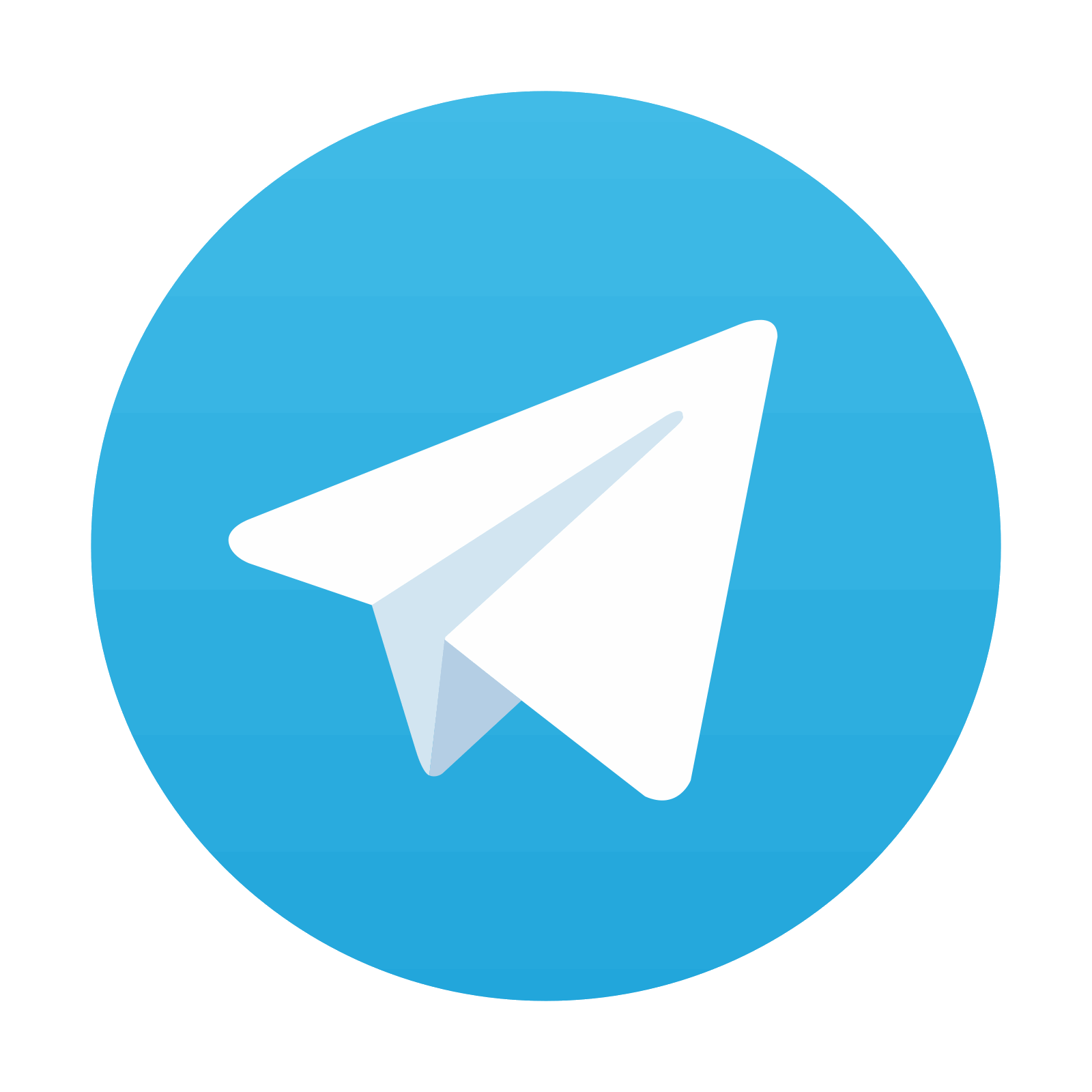
Stay updated, free articles. Join our Telegram channel
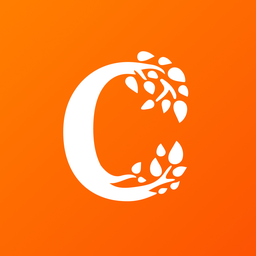
Full access? Get Clinical Tree
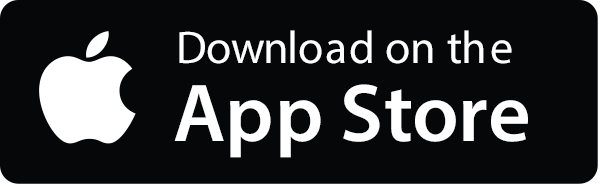
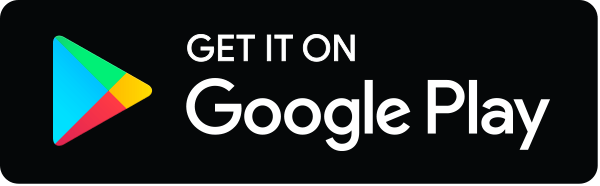